APPENDIX D Energy and Power Materials
A NOTE ON POWER SOURCES
There is a continuing need for materials that can store large amounts of energy in small packages and use the energy efficiently. In materials terms, advanced energy storage requires materials that have high volumetric and gravimetric energy densities. While many of the approaches discussed in this report can affect several types of power sources, it is important to realize that no single power source is appropriate for all applications (see Figure D-1).
A range of energy storage and conversion technologies will have central roles in engineered energy storage and delivery systems for powering DoD needs. High-energy needs are likely to be met with a liquid fuel because the energy density of liquid fuels, in reaction with air in an internal combustion device or fuel cell, is unsurpassed. High-power applications, on the other hand, are likely to require batteries, or more likely, hybrid power devices that combine a high-energy system (e.g., a fuel cell) with a high-power system (e.g., a powerful battery or electrochemical capacitor). Properly designed, a system can enjoy the benefits of both the high energy content of a liquid fuel and the high power of a battery.
In the main body of this report, the committee attempts to show that some material improvements can affect several technologies. Batteries and fuel cells will both benefit from improved electrolytes and better methods to tailor electrochemical interfaces. Other improvements will affect one technology more than another or may even be specific to a single technology.
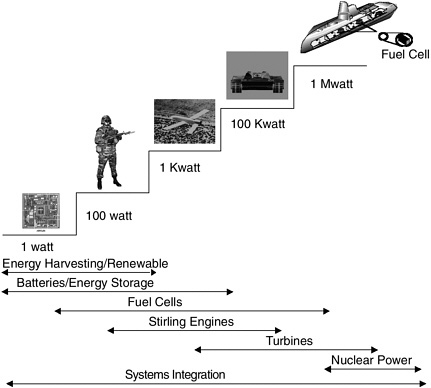
FIGURE D-1
Military systems power requirements often follow a “step function,” so different power sources are needed for different applications. SOURCE: Carlin, Richard, ONR, Electric Power Sources for the Navy and Marine Corps, Presented at the Navy Grand Challenges Workshop, November 16-18, 1999.
COMMERCIAL VERSUS MILITARY BATTERY REQUIREMENTS
Many specific military requirements limit the use of commercial off-the-shelf (COTS) power sources. These include differences in required power and energy levels, in temperature operating range, in the need for shock resistance, in longevity requirements, and in reliability levels. Except in hybrid electric vehicles (HEVs), high-power batteries are generally not needed in the commercial marketplace, and even HEVs are not subject to the same performance demands that future defense applications will encounter. Materials for military use must also operate in a broader temperature range and under more rigorous environmental extremes than materials for commercial use. Military batteries must have a longer shelf life and lower self-discharge rates than typical commercial batteries.
Furthermore, commercial devices are not held to the same reliability standards as military devices. The reliability driver for commercial power sources is to minimize warranty expenses; the reliability driver for future defense systems is mission success and soldiers’ lives. These differences, which are present in all the Services, are typified in the requirements for Navy batteries shown in Figure D-2.
DIELECTRIC ENERGY STORAGE
Capacitor dielectrics will continue to have an important role for DoD, particularly for energy storage, power conditioning, high-rate switching, and pulsed power for a range of future DoD systems. Compared to other forms of electrical energy storage, capacitors are lower in energy density
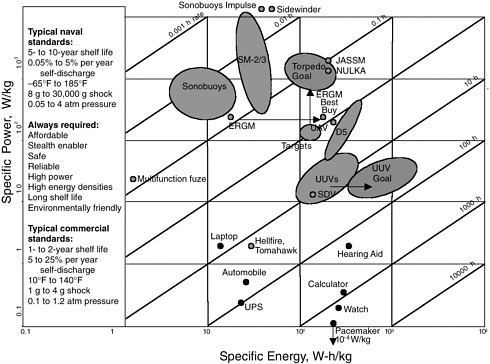
FIGURE D-2
Military versus commercial requirements for batteries. SOURCE: Suddeth, David, Naval Surface Warfare Center, Managing Power Source Strategies for Navy Applications, Presented at Navy Conference in Crystal City, VA, December 11-13, 2001.
but higher in power density (see Figure D-3), and they are more readily cycled at high frequencies.
EXPLOSIVES AND PROPELLANTS
Explosives
Many of the key chemical explosives of military interest today were first synthesized in the late 1800s and, as with structural materials, moving from laboratory curiosity to general application of energetic materials has often taken 20 to 30 years, as shown in Table D-1 (Federoff, 1960). Important technological parameters for high explosives are energy release/ reaction propagation rate; energy density; and resistance to accidental explosion (insensitivity). Increased insensitivity to accidental detonation
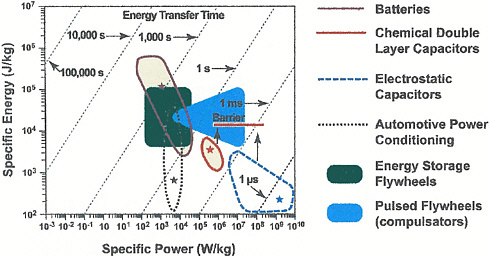
FIGURE D-3
Power versus energy density for selected mechanisms for electrical energy storage.
SOURCE: Clelland, I., R. Price, and J. Sarjeant. 2000. Advances in Capacitor Technology for Modern Power Electronics. Pp. 145-148 in Proceedings of the 24th International Power Modulator Symposium, Norfolk, VA, IEEE, Piscataway, NJ.
TABLE D-1 First Synthesis of Chemical Explosives of Military Interest
has been a major focus of modern explosives research over the past 40 years, and efforts continue to reduce sensitivity while increasing explosive energy.
Over the last 50 years, the average power of military high explosives has increased by approximately 40 percent. All indications are that tomorrow’s weapon systems will be smaller than today’s. Unless they are to carry a disproportionate share of mass in weapons and explosive power, achieving the same energy on target will require higher energy density materials (HEDM).
Is there room for additional improvement? Figure D-4 depicts the mass-based energy density spectrum (cal/gm) and the relative position of conventional high-energy chemistry within it. Conventional hydrocarbons based on oxygen and nitrogen (CHNO) explosive materials are at the low end, on the order of 103 cal/gm. New CHNO-based molecules combined with advanced approaches for achieving high surface areas and mixing components may be able to increase this figure several-fold—the equivalent of several centuries of progress. The figure indicates that there may be even much greater potential for HEDM based on alternative approaches. Some of the latter are currently only at the conceptual stage; others are just entering the basic research stage.
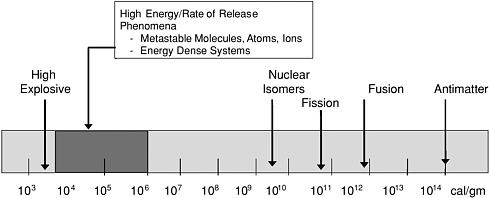
FIGURED-4
Is there room for improvement for energetic materials? Energy density per unit mass.
SOURCE: Ullrich, G.W., director, Weapons Systems, Office of the Secretary of Defense, Advanced Energetic Materials: Introduction and Overview, presented to the Committee on Advanced Energetic Materials and Manufacturing Technologies, National Research Council, Washington, DC, July 31, 2001.
Propellants
Propellants burn without exploding and contain all the oxygen they need for combustion (Alchavan, 1998). Ideal propellants are dense; occupy little space before burning; produce only low-molecular-weight gases and heat; show repeatable performance; yield acceptably low gas temperature and products to reduce gun damage; provide maximum gas output within the pressure limits of the gun; resist shock, impact, and heat; are safe to store and handle; and are low-cost and environmentally acceptable. Specific impulse (Isp) is one of the performance measures most often used for propellants. An additional measure of efficacy is the amount of oxygen needed to convert all oxygen to carbon dioxide and all hydrogen to water (Cooper and Kurowski, 1996). Propellants emit gas and heat but, in contrast to explosives, do not react faster than the bulk sound speed in the material.
Traditional propellants (single-, double-, or triple-base materials) are composed of CHNO molecules that self-oxidize; they have historically been used in guns or small military rocket motors. In a separate class of materials is the composite propellant, which contains fuel and oxidizer as
separate components, and which is used extensively in rockets and for gas generators other than in guns. The fuel often acts as a binder for the components. Although both liquids and solids have been employed as rocket/missile propellants,1 all major gun propellants today are solid.2 This report concentrates on opportunities in solid propellants because of their widespread use across DoD and their similarities to explosives.
Key Issues for Energetic Materials
Much effort over the past few decades has been directed to safety and process improvements in explosives and propellants. In particular, creating munitions insensitive to accidental detonation has been a major goal. Traditional approaches to development of insensitive materials for explosives and propellants have relied quite heavily on intuition. This process is time-consuming, with no guarantee of success. To date, there has been relatively little application of atomistic modeling to energetic materials formulation. The problem has been recognized by issuance of a Defense Strategic Research Objective (SRO) on Insensitive High-Energy Materials.3 A new paradigm is slowly evolving in which science-based models are supplementing, and in some cases replacing, intuitive approaches to munitions design. One estimate is that development time for propellants using current ingredients requires 10-15 years, whereas development of new materials and the associated formulations can require 25-40 years,4 in part because empirical testing requires use of multiple iterations at multiple scales. An important goal is to reduce this development time.
The development of novel energetic materials over time has relied on the intuition, ingenuity, and knowledge of experienced individuals and on extrapolation of the properties and structures of existing energetic materials to those of new materials. These complex materials will continue to be a fundamental contributor to U.S. national defense in the future.
To streamline the R&D process in this area, effort should be directed at assembling and integrating the entire arsenal of materials development capabilities. These include use of (1) computation materials science in materials design to help identify promising new molecules and formulations; (2) new material synthesis approaches; (3) improved processing methods; (4) new characterization techniques; and (5) advanced modeling to reduce the expensive and time-intensive testing necessary to verify performance.
Finding advanced approaches to computation materials science, combining first principles and known design rules with the results of well-designed experiments, has the potential for reducing the amount of time required to identify, develop, and verify the performance of new energetic materials. Already, molecular modeling techniques applied to the development of new energetic materials are showing promise of materials that provide enhanced energy density and insensitivity.
It is important to understand the fundamental processes for initiation of energetic materials and, in the case of propellants, the associated fundamental combustion mechanisms. Having a comprehensive model of reaction processes in heterogeneous nanoscale energetic materials, taking into account details of initiation and propagation of reaction fronts, would help us understand these complex processes and would form a basis for design and development of novel energetic materials.5
Many of the materials that have already been identified as promising have no known synthesis routes. Others can be prepared, but only in gram quantities by costly means requiring either multistep synthetic approaches, expensive reagents, difficult-to-maintain experimental conditions, or combinations thereof. Making novel energetic materials available in large quantities for military use will require improved means for synthesis and
new processes for combining these materials with others in complex formulations.
As is discussed below, one promising avenue to new energetic explosives and propellants is to use nanomaterials technology and nanocomposite materials. In parallel with developments in the rest of this emerging field, this will require new techniques for characterizing these materials at different size scales, particularly the nanoscale. Of particular interest in these nanocomposite energetic materials are such factors as nanoparticle size, size distribution, morphology, surface chemistry, and composition. These variables have all been shown to be important in determining mechanical, barrier, and other properties of nanocomposites (Kornmann et al., 1998; Messersmith and Giannelis, 1995), and they will likely control the contributions of nanoscale components in energetic materials as well.
Finally, the time required to field new energetic materials is long, due in no small part to the rigorous testing required to assure their performance, reliability, and safety. Again, use of new computational approaches may help compress this time schedule.
LOGISTIC FUELS
An overriding military concern in fuels technology is logistics. Fewer fuels mean a simplified logistics burden and the ability to provide energy, rapidly and flexibly, to the system that most requires it. From this perspective, all military systems would ideally run on a single fuel.
Many current military propulsion systems have been designed to use a variety of petroleum-based fossil fuels, such as jet fuel (e.g., JP-5, JP-8) and diesel. These fuel families are exceedingly well-established in military applications and are supported by a strong logistics infrastructure. The panel recognizes that new electrochemical propulsion and power systems employing fuel cells are in various stages of design and development and it believes that their development as outlined in Chapter 4 is essential for the DoD applications of 2020.
However, it also appears that current hydrocarbon military fuel families (jet fuel and diesel fuel) will continue to power most military platforms in 2020 due to their established position as workhorse fuels, combined with the cost and time required for propulsion system redesign to produce other fuels. Convergence to use of a single petroleum-based
logistic fuel is also unlikely because diesel fuel, now used in a variety of trucks and military ground vehicles, will not flow readily in a jet engine, while gasoline is too volatile. Therefore, while there is a strong push toward novel fuels for fuel cell applications, in 2020 fuels for turbine and internal combustion engines used to transport men and materiel are expected to remain primarily hydrocarbon-based.6 Reformed diesel and jet fuels are also likely to retain a prominent role even when fuel-cell-based systems arrive in large numbers, due to the strong established base for these fuels.
These conclusions are supported by examination of fuel-related developments in the commercial sector, which shows a continued push to improve petroleum-based fuels and power sources. In 2020, oil and natural gas are expected to remain the dominant energy supply for the United States, with civilian transportation fuels obtained primarily from hydrocarbons. Production of petroleum-based fuels is expected to peak between 2020 and 2040. Throughout the transportation sector a global shift to cleaner fuels is expected by 2020, raising energy efficiency and reducing greenhouse gas emissions. Concurrent efforts will be made to improve civilian transport efficiency by way of leaner burning internal combustion systems, hybrid electric vehicles, fuel cells, greater use of mass transportation, and telecommuting. The environment will drive changes in petroleum-based fuel formulation to reduce volatile organic compounds, NOx, particulates, and greenhouse gas emissions. Most industrialized nations will continue to seek reductions in SOx emissions in jet fuels while retaining fuel lubricity and performance. In the civilian sector, hybrid internal combustion/electric drives and other novel drive systems will evolve for automotive engines. Power sources in civilian automotive applications will include both petroleum fuels for Carnot cycle engines and hybrid gas-electric systems, with portions of the market being filled by approaches such as all-electric power, compressed or liquefied natural gas, or hydrogen. Because these latter approaches will need considerable new infrastructure to be effective, their widespread adoption is likely to be delayed.
Improvements to today’s logistic fuel technology are expected to be largely evolutionary. While many of the problems are not materials-related
per se, their solutions could lead to improvements in military fuels. Modifying refinery yield to improve fuel quality (e.g., by reducing sulfur) or to optimize yield of one particular fuel fraction is one such evolutionary approach. Another is fuel interconversion using ring-opening reactions. These can convert cycloaliphatics or aromatic compounds to branched aliphatics. This would facilitate conversion of various components of diesel fuel to jet fuels of different types. Accomplishing this would require improved catalysts. The technology appears to be feasible, but there is no real economic incentive to accomplish it in the civilian market.
Changing the scale of a process to provide higher heat and mass transfer or to optimize yield is a developmental approach that appears to be predominantly a chemical engineering challenge. However, at the lower end of the size scale, it devolves into solving materials-related engineering problems because the size of components must become quite small. Here, large numbers of MEMS or mesoscale processors are used in parallel to improve yield, reduce emissions, provide more rapid heat and mass transport, enhance reaction rate, and improve safety. This microscale process intensification approach could result in revolutionary rather than evolutionary processing capabilities if the design and manufacturing difficulties associated with combining large numbers of very small processors and components into a single practical system can be overcome.
In summary, for logistic fuels most changes through 2020 will remain evolutionary. Potentially revolutionary advances in fuels will come from using micro/MEMS-based chemical approaches to processing logistic fuels. Other advances that relate to methods for on-board reforming of logistic fuels and for storing fuels like hydrogen are discussed in the section on fuel cells in Chapter 4.
FUEL CELLS
Description
Fuel cells are devices that directly convert chemical energy into electrical energy and, because they do not involve combustion, are not Carnot-limited. The principle of operation of a fuel cell is illustrated in the text of the Energy and Power Materials Panel report (Chapter 4). Much as in a battery, the components in a fuel cell that make direct electrochemical conversion possible are an ion-conducting electrolyte, a cathode, and an
anode. Fuel is fed to the anode and an oxidant to the cathode. Oxidation of the fuel proceeds electrochemically via “half-cell” reactions that take place at each electrode. The rate at which these reactions occur, and thus the efficiency of the fuel cell, depends on the performance of the catalysts incorporated into the electrodes. Fuel cell efficiency is also directly related to the area-specific conductivity (conductivity/thickness) of the electrolyte. In the case of liquid electrolytes, the electrolyte is infused into some sort of matrix or sponge material, the surface properties of which are optimized to retain the corrosive electrolyte liquid within its structure.
Several different types of fuel cells have appeared over the past few decades; they are differentiated essentially by the type of electrolyte they employ (Table D-2), which in turn determines the temperature at which the fuel cell is operated. For efficiency purposes, higher temperature operation is preferred, but for portable power applications, lower temperature operation is preferred. High temperatures, as employed for molten carbonate and solid oxide fuel cells, generally ensure that reactions are rapid and a variety of hydrocarbon fuels can be used directly in the fuel cell. However, start-up times can be very long, and there is a limited number of (usually expensive) materials available for fabricating the fuel cell. Low
TABLE D-2 Fuel Cell Types and Selected Features
Type |
Temperature °C |
Fuel |
Electrolyte |
Mobile Ion |
Recycled Species |
Polymer electrolyte membrane (PEM) |
70-110 |
H2, CH3OH |
Sulfonated polymers (Nafion™) |
(H2O)nH+ |
H2O |
Alkali fuel cell (AFC) |
100–250 |
H2 |
Aqueous KOH |
OH– |
H2O |
Phosphoric acid fuel cell (PAFC) |
150-250 |
H2 |
H3PO4 |
H+ |
|
Molten carbonate fuel cell (MCFC) |
500-700 |
Hydrocarbons, CO |
(Na,K)2CO3 |
CO32– |
CO2 |
Solid oxide fuel cell (SOFC) |
700-1000 |
Hydrocarbons, CO |
(Zr,Y)O2– |
O2– |
|
SOURCE: Hirschenhofer et al. (1998); Larmine and Andrews (2000); and Williams, M., “Overview of Fuel Cells,” briefing presented to the Panel on Energy and Power Materials of the Committee on Materials Research for Defense After Next, National Research Council, Irvine, CA, October 10, 2001. |
temperatures, as employed in proton exchange membrane fuel cells, are advantageous for intermittent power applications because start-up times are short, but at low temperatures chemical reactions are slow and fuel choices are essentially limited to hydrogen and possibly methanol.
While there are several technological hurdles to be surmounted before fuel cells become commonplace in either the civilian or military sectors, they offer several attractive features. In particular, (1) they are highly efficient, easily 45 percent for high-temperature fuel cells with even greater efficiencies in hybrid systems; (2) because of their operation on hydrocarbon fuels, they offer the potential for very high energy and power densities; (3) they are easily scaled, without significant loss in performance parameters, to a wide range of power demands; (4) they are nonpolluting (NOx and SOx emissions are ultralow); (5) they can be implemented in a distributed, modular fashion, resulting in a power generation system that is robust under combat conditions; and (6) they can be operated in a closed-cycle system that uses as input solar energy or fuel cell waste products to generate chemical fuels suitable for reuse in the fuel cell. This mode of operation is particularly advantageous for remote military operations, in which reliance on delivery of fuel implies a significant vulnerability.
Each of the fuel cell types listed in Table D-2 has been demonstrated in complete fuel cell systems, with AFC and PAFC being the most mature technologies and SOFC and PEMFC the newest. The choice of electrolyte places fundamental constraints on fuel cell system design. First, if a liquid electrolyte (alkali, phosphoric acid, or molten carbonate) is used, there must be some method of containing the electrolyte, which is corrosive to most materials, yet ensuring its access to fuel and oxidant gases. Second, if the ion transport relies on a “vehicle” mechanism—carbonate ions to shuttle oxygen (MCFC), hydronium ions to shuttle protons (PEM), or hydroxyl ions to shuttle oxygen (AFC)—then the fuel cell system inherently requires recycling of an inert gas—CO2 in the first case or H2O in the other two cases. Third, if we compare “true” proton conductors (not hydronium ion conductors) and oxygen ion conductors, both result in equally simple fuel cell systems, so long as the fuel is hydrogen, but for hydrocarbon fuels, SOFCs offer simpler systems because oxygen is supplied to the fuel and complete reaction to form CO2 and H2O is in principle possible. In the PEM system, protons are “stripped” from the fuel; to avoid carbon deposition, additional water must be fed to the anode to encourage reforming of the fuel (usually methanol) into CO2 and H2. Thus, oxygen ion-conducting
electrolytes offer the potential for extremely simple fuel cell systems, although in practice, this has not been achieved.
Demonstrated fuel cell system efficiencies are in the range of 40 to 45 percent, and much higher efficiencies are expected when waste heat recovery schemes are used. Today’s system costs range from ~$4,500/kW for phosphoric acid and molten carbonate fuel cells to over $10,000/kW for solid oxide, polymer electrolyte, and alkali fuel cells. In the Partnership for the Next Generation of Vehicles program (now abandoned), PEMFC costs were targeted to drop to $50/kW by 2004. DOE analyses predict that costs of ~$800/kW can be reached in solid oxide systems by 2005.7
Fuel Cell Research Efforts Required to Meet DoD Needs
This section expands on the breakthroughs needed in fuel cell research identified in the main body of the report.
Electrolytes and Ion Transport
A key limitation of fuel cell performance for all types of fuel cells is the resistance of the electrolyte, particularly in solid oxide fuel cells, for which high resistivity requires high-temperature operation, which in turn requires use of costly materials for the other fuel cell components. Research efforts to enhance the ionic conductivity of oxides and thus enable reductions in operating temperature (~600°C) are under way at many university and government laboratories. These efforts have yielded new materials like (La,Sr)(Ga,Mg)O3 (LSGM), an oxide ion conductor, and doped BaCeO3, a proton conductor, leading to optimization of known materials like doped CeO2, an oxide ion conductor. So far, few studies of these materials as electrolytes in single-cell fuel cells have been carried out; presumably, their high conductivities will translate directly into increased fuel cell efficiencies, but little is known about the appropriate electrocatalysts for such systems. Given the broad range of oxide materials already known but unexamined as electrolytes, and those yet to be discovered, the potential for uncovering materials with enhanced ionic conductivity, excellent chemical stability, and good mechanical integrity is tremendous.
Polymer electrolyte membrane fuel cells rely on relatively facile transport of hydronium ions through hydrated regions of the sulfonated polymer. Their high conductivity allows for operation at close to ambient temperatures. The hydrated nature of the polymer electrolyte gives rise to some daunting challenges: (1) because the mobile species is hydronium, high humidification is required to maintain high conductivity, yet this must be balanced by the need to remove water from the cathode and prevent flooding of the electrocatalyst, (2) the maximum temperature of operation is ~100°C, (3) methanol diffuses from anode to cathode in a direct methanol PEM fuel cell, and (4) mechanical properties degrade due to “swelling” upon hydration. As with SOFCs, a broad-based research effort is under way to develop polymeric materials that do not suffer from these drawbacks. Indeed since 1995, the automotive industry has invested ~$2 billion worldwide in PEM fuel cells, with a significant portion of that investment directed at improving membranes. In many cases, however, success in, for example, reducing methanol crossover has come at the price of reduced conductivity. A radically different approach is to use anhydrous inorganic proton conductors based on acid salts (e.g., CsHSO4) that truly transport protons rather than hydronium ions. While this approach has demonstrated success, limitations due to electrolyte solubility in water and degradation under fuel cell operating conditions are yet to be addressed (Haile et al., 2001).
In the case of molten carbonate, alkali, and phosphoric acid fuel cells, it is fair to conclude that further significant advances in the electrolyte material, particularly in ionic conductivity, are unlikely. Advances are more likely to come about through enhancements in the stability of the matrix material (through modifications to both the electrolyte and matrix compositions) and via processing routes that result in matrix structures with well-defined and reproducible porosities and surface areas. These advances are considered evolutionary rather than revolutionary.
Exploratory synthesis of new solid-state electrolyte materials, guided by computational materials science and enhanced by combinatorial approaches, thus constitutes an area ripe for producing revolutionary advances in fuel cells for future defense systems. Solid-state conductors of either oxide ions or protons with conductivities of ~10–3 S/cm at temperatures between 25 and 500°C and sufficient mechanical and chemical integrity would completely alter the fuel cell landscape. Direct methanol fuel cells (DMFCs) are a good example. Because they are relatively simple and have few auxiliary components, they are considered most suitable for
single-soldier applications. In today’s DMFCs, power densities are low because the methanol concentration in the fuel, a mixture of water and methanol, is limited to no more than ~3 percent and the electrolyte is relatively thick. Higher concentrations and thinner electrolytes lead to dangerous levels of methanol crossover (diffusion across the hydrated polymer), which can cause uncontrolled combustion. Zero methanol crossover electrolytes would reduce the amount of unnecessary water carried by the soldier by a factor of about four while increasing the power density of the fuel cell by at least a factor of two. Though civilian applications would also benefit from such electrolytes, the option of alternative fuel cell types in many of those applications (e.g., reformate-based PEMFCs) lowers civilian motivation to address this challenge. In sum, DoD investments in perovskite and fluorite oxide ion conductors, perovskite proton conductors, and anhydrous acid salts as pathways toward revolutionary new electrolyte materials are warranted.
Electrodes and Electrocatalysis
Critical to the function of a fuel cell are the electrodes and electrocatalysts, which catalyze the electrochemical reduction of the oxygen at the cathode and the oxidation of the fuel at the anode. The relatively poor performance of today’s anode catalysts necessitates the use of hydrogen, or possibly methanol, as the fuel in lower temperature fuel cells (PEM, PAFC, and AFC). Indeed, in PEM fuel cells the hydrogen must be free of impurities in order to ensure proper catalyst performance (<50 ppm CO and <50 ppm H2S).8 The low operation temperatures of these fuel cells, along with the chemically aggressive nature of the electrolyte, further limit electrocatalyst choices to costly precious metals, except in alkali fuel cells. Inadequate anode catalyst performance is also what limits the use of diesel fuels in higher temperature (SOFC and MCFC) fuel cells, although clean alkanes (methane, ethane, propane, etc.) can be readily used. Poor cathode catalyst performance lowers fuel cell power densities, thereby making necessary larger and more costly systems, but generally does not affect the choice of fuel.
Breakthroughs in fuel cell electrocatalysts would revolutionize fuel cell technology, but it is not at all clear how such breakthroughs can be
achieved. Extensive research, from fundamental studies of H2 adsorption on Pt single crystals to Edisonian “try-and-tinker” approaches to new catalyst alloys, is currently under way in laboratories worldwide. Some of these efforts have already led to important changes in our understanding. Two are highlighted here.
It has long been believed that the use of hydrocarbon fuels in solid oxide fuel cells requires an internal reforming reaction step, in which water and fuel react in the vicinity of the fuel cell anode to produce CO2 and H2, and that the hydrogen so generated is then consumed electrochemically to produce electricity. Groups at Northwestern University (Murray et al., 1999) and the University of Pennsylvania (Park et al., 2000) have independently shown that, in fact, water is not required for fuel cell operation. These results have radically changed the picture of anode reaction pathways and suggest that simpler fuel cell designs in which water recirculation is not necessary are on the horizon.
Perhaps even more significant have been reports from the Industrial Research Institute of Nagoya, Japan, indicating that fuel cell reactions can be so well controlled by the electrocatalysts that fuel and oxidant need no longer be separated (Hibino et al., 2000). The simplifications that might be afforded by such a “single chamber” fuel cell system are breathtaking. It is critical that the reliability of these results be verified by independent laboratories.
The importance of electrocatalysts, and the absence of a clear pathway for success, dictate that the military explore a broad range of solutions to address the challenge of improving fuel cell catalysts, particularly diesel fuel anode catalysts, which are of lesser interest to the civilian sector. Many tools developed in the last few years can and should be effectively leveraged by DoD as part of this effort: (1) combinatorial chemistry methods for quickly finding and optimizing new alloy compositions for catalysts, (2) computational materials chemistry for guiding exploratory research in identifying new catalysts, and (3) new synthetic methodologies that allow for architectural control of fuel cell electrodes at the nanoscale, potentially enhancing reaction kinetics by dramatically increasing electrode surface area and restricting reactions to confined regions. The fuel cell community has yet to take advantage of advances in these areas, and it is at the interface between these fields and traditional electrochemistry that the most significant breakthroughs can be anticipated.
Fuels and Fuel Cell System Design
It is evident from the previous discussion that the choice of fuel for fuel cell systems is a fundamental question. While higher temperature fuel cells can operate directly on hydrocarbon fuels (typically using water to achieve internal reforming), lower temperature fuel cells operate on hydrogen or possibly methanol. Generating, storing, and transporting hydrogen is a significant hurdle. Hydrogen is typically produced from methane (CH4), which is reacted with water (reforming) or oxygen (partial oxidation) at high temperature to produce a mixture of CO2, CO, H2, and residual CH4. The CO in this mixture is then reacted with H2O at lower temperatures to yield CO2 and H2 via the “water gas shift.” Further reductions in CO concentration can be achieved by preferential oxidation (reaction with oxygen again).
Where the starting material is some other fuel, sulfur removal is also an important step. Removal of trace levels of CO is of paramount importance for PEM fuel cells, whereas trace levels of sulfur are problematic for all fuel cell types. These impurities adsorb onto the surface of the anode catalyst, reducing fuel cell voltage and thus power output and efficiency.
Today’s fuel reformers are extremely efficient (~85 percent) because of tight thermal integration and effective catalysts but do not produce H2 pure enough for long-term PEM fuel cell operation. Methane reformers are the most mature technology; gasoline reformers are under rapid development in the civilian automotive sector. For DoD applications, reformers that process diesel fuels for lower temperature fuel cells or high-temperature fuel cells that use diesel fuels are essential. Avoiding dependence of the military on imported fossil fuel sources may require effective strategies for coal gasification and subsequent hydrogen generation, as well as effective methodologies for sequestering CO2, the natural byproduct of any energy production involving coal.
In recent years, there have emerged new approaches for generation of clean hydrogen from a variety of fuel sources that warrant further DoD investment. These rely on membrane reactors in which the reactants (for example, CO and H2O in a water gas shift reactor) are fed into one side of a membrane that selectively transports one species (hydrogen in this example); the reaction products are then extracted from the other side of the membrane (Figure D-5). The effect of the water gas shift reaction is to both ensure that pure hydrogen is generated (no other species can transverse the selective membrane) and enable hydrogen production rates
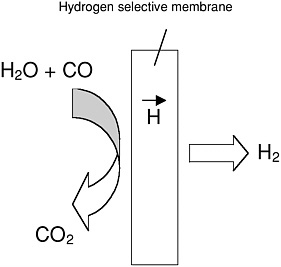
FIGURE D-5
Schematic of a membrane reactor, using the water gas shift reaction as an example. The overall reaction is H2O + CO → H2 + CO2. The right-hand side of this reaction is favored by rapid removal of H2 from the reaction zone.
beyond the thermodynamic limit, because the hydrogen concentration in the reaction regime is kept very low. Successful operation of membrane reactors requires materials with high hydrogen fluxes, good catalytic properties, and excellent chemical and mechanical robustness. Research in this area is in its infancy, with most of it directed at palladium-based membranes and some limited work on ceramic membranes. Given the potential of such reactors to solve the daunting fuel problem for fuel cells, additional efforts in this direction are needed.
An entirely different strategy for providing hydrogen for fuel cells is the use of nonconventional fuels like ammonia, hydrazine, or sodium borohydrate. As with hydrogen, another energy source must be used to synthesize these fuels, but they offer the advantage of being processable in a closed cycle, so that reliance on fossil fuels is minimized. Particularly attractive is sodium borohydrate, which readily reacts with water to release hydrogen and the byproduct, sodium borate, can be regenerated using independent energy inputs.
INORGANIC THERMOELECTRIC MATERIALS
Thermoelectric (TE) materials have applications like thermal management, man-portable cooling, and recovery and conversion of waste heat
into useful electrical power, all of which are of importance to DoD. Cooling devices made from these materials contain no moving parts or environmentally unacceptable working fluids like chlorofluorocarbons. Such devices can have high reliability, low weight and volume, and low acoustic signature while allowing for precise temperature control.
TE modules are based on materials with a unique combination of properties, including high thermopower but also high electrical conductivity combined with low thermal conductivity. This combination of properties is critical to high device efficiency but is difficult to achieve in a single material, because these properties are often quite interdependent, with (for example) high electrical conductivity often connoting high thermal (phonon) conductivity as well. This situation is both a great challenge and an exciting opportunity for solid-state chemists, theorists, and materials scientists to devise creative approaches to optimize all the desired properties.
The figure of merit for a TE material is defined as ZT = (S2σ/k)T, where S is the thermopower (or Seebeck coefficient), σ the electrical conductivity, κ the thermal conductivity, and T the temperature. When a temperature gradient is applied to a thermoelectric material, the mobile charge carriers at the hot end preferentially drift to the cold end, inducing a voltage; this is called the Seebeck effect. The sign of the Seebeck coefficient depends on the sign of the charge carriers. Consequently, a pair of n-type and p-type “legs” can be placed in parallel with a temperature gradient but in series with respect to electron flow and the voltage generated across each leg will be additive; power is delivered to a load placed in the circuit from both legs. Useful TE materials must not only exhibit high ZT over a wide temperature range but must also be compatible with both donor and acceptor doping. Although the two legs could be made of unrelated n- and p-type compounds, complications with material compatibility and device design can arise if their properties (coefficient of thermal expansion, thermal conductivity, electrical conductivity, etc.) differ significantly.
While TE devices are highly desirable, they are not widely available due to their low efficiencies, a fact directly attributable to the low values of ZT in known materials. Even the best TE materials exhibit bulk values of ZT that are only on the order of 1, a milestone that was achieved nearly 40 years ago (Vining, 2001). For viable TE devices for DoD, values of ZT up to 4 are necessary. Such targets, while they have remained elusive, violate no laws of thermodynamics. Indeed, there is no fundamental limit to the possible value of ZT, so the slow progress of recent years calls for new approaches to TE materials.
The best materials are based on complex semiconductors with optimized carrier concentration, high effective mass carriers, high carrier mobility, narrow bandgap, and low lattice thermal conductivity (Nolas et al., 2001). Examples are Bi2Te3, PbTe, Si0.8Ge0.2, and Zn4Sb3. Unlike many technological solid-state electronic materials, in which simplicity in composition and structure and extreme crystalline order and purity are sought, TE materials are more likely to have complex composition, elaborate crystal structure, and even disorder, all features that may lower lattice thermal conductivity without sacrificing electrical conductivity and thermopower. Moreover, a single material is unlikely to exhibit high ZT over the entire temperature range of interest. Therefore, a broad-based and sustained exploratory synthetic effort that encompasses many classes of materials and structures (chalcogenides, pnictides, intermetallics, oxides, etc.) and even leads to entirely new families of materials must be pursued if the challenge of high ZT is to be met. Here solid-state chemistry is of central importance.
Nanoscience may also have a role to play in next-generation thermoelectrics. Certain theoretical considerations predict that quantum confinement, which can increase the density of states at the Fermi level, may be another path to systems with high ZT. Quantum confinement using multilayered structures can also provide between-layers interfaces that selectively scatter or confine phonons (lattice vibrations) to reduce thermal conductivity. Thin-film TE materials and devices thus offer exciting possibilities. Preliminary results for Bi2Te3/Sb2Te3 superlattices are very promising, with a reported effective ZT of 2.4 (at room temperature) (Venkatasubramanian et al., 2001); efforts must now be directed toward implementing such structures in functional devices to experimentally verify this concept.
Any exploratory synthetic effort toward new TE materials must be complemented with a strong computational/theoretical effort, particularly in electronic band structure calculations and interpretation. Several parameters are critical in establishing TE behavior: actual bandgap, the shape and width of the bands near the Fermi level, the carrier effective masses and mobilities; and the degeneracy of band extrema (i.e., the number of valleys in conduction band or peaks in the valence band). Of the three properties that define Z, thermopower is least understood. It cannot be predicted, nor is it known how to increase the thermopower of a material without depressing electronic conductivity. In enhancing understanding, solid-state theory can provide invaluable guidance in selecting
materials for consideration. The synthetic effort must also be matched by breakthroughs in approaches and methods of screening large numbers of candidate materials reliably and quickly. This necessarily brings together the fields of solid-state chemistry, solid-state physics, materials science, and electrical engineering. Close collaboration between scientists from these areas is of paramount importance.
INORGANIC PHOTOVOLTAICS FOR ENERGY CONVERSION
Organic photovoltaic (PV) materials are discussed in Chapter 6. This section briefly addresses materials and processes for devices made from inorganic materials that are useful in converting light into electricity. These devices offer the potential for harvesting ambient solar energy and are of interest because the input energy is free and the process is nonpolluting. If sufficiently high efficiencies could be obtained, these devices would be revolutionary for DoD in simplifying logistics and modifying energy resupply requirements. In theory, solar radiation could supply electricity and also make it possible to produce hydrogen from available water to fuel hydrogen fuel cells. Even at lower efficiencies, these devices could provide some portion of the energy a soldier needs in combat.
PV devices convert light directly into electricity with no net chemical change left behind. They have been based on such materials as doped forms of single-crystal, polycrystalline, or amorphous silicon; compound semiconductors have been based on III-V materials. An alternate type is a photosynthetic cell with two redox reactions, one involving reaction with electrons at the counterelectrode and one with holes at the semiconductor electrode. This type of cell, also known as a photoelectrochemical (PEC) cell, can be used to generate electricity from light or can convert light into chemical potential energy via photoelectrolysis. While TiO2 has been used successfully as a semiconductor in PEC cells, it absorbs primarily in the UV portion of the spectrum and has low conversion efficiency. Use of the cubic perovskite SrTiO3 raises conduction band edge energy and allows spontaneous photoelectrolysis of water, which has been one objective of photoelectrochemists for years. However, the material still functions in the UV and efficiency remains too low for commercial use. Attempts to produce other oxides that are environmentally robust and that absorb preferentially in the visible range have been largely unsuccessful. Separation of the functions of optical absorption and charge generation has led to improved devices. This has been achieved by combining oxide semi-
conductors with ruthenium-based sensitizing dyes that absorb in the visible and facilitates injection of charge carriers into a stable (wide bandgap) semiconductor (Hamnett and Christensen, 2000).
Nanomaterials offer potential dramatic property improvements, here in terms of devices with higher efficiencies and enhanced environmental stability. Examples are dye-sensitized mesoporous film oxides or chalcogenides, which are composed of nanometer-sized crystals, are dye-sensitized, and have their porosity filled with a second medium that can be solid or liquid and that involves a semiconductor or a conducting electrolyte. The extremely large contact area when combined with dye sensitization can lead to much enhanced (1,000 times) capture of incident light and greatly increased photocurrents. Similarly, solid heterojunctions having large contact area can be grown from electron and hole-carrying materials in the form of interpenetrating networks and have demonstrated some promise (Grätzel, 2001). Future effort in this area should examine nanoscale structures like semiconductor quantum dots for efficient photon collection and rapid charge conduction, with a goal of increasing device efficiency while reducing manufacturing cost. This cost has slowed the adoption of PVs in the past and is one reason (together with low weight and ability to employ flexible substrates) that effort has been directed toward organic PVs. If PV approaches are to become feasible for DoD or the commercial arena, a low-cost means of making PV cells in large quantities is essential. Today’s laboratory-scale techniques for generating promising material configurations must give way to truly manufacturable approaches.
ELECTRIC POWER GENERATION FOR FUTURE DOD SYSTEMS: EFFECTS OF ELECTRIC PROPULSION IN SHIP DESIGN
Platform propulsion and support using more electric systems is of importance to the Army, Navy, and Air Force. The consumption of electric power by elements of DoD will likely increase significantly over the next 25 years. More correctly, the ability to consume power will rise; with better efficiency, the actual increase in consumption can be mitigated, though several factors will push the overall electrical load higher. Using a U.S. Navy ship as an example, this section (1) summarizes why electric power use may increase; (2) shows how integrated electric propulsion might positively affect ship design; (3) identifies specific DoD technology needs;
and (4) briefly points out materials issues. The goal of this appendix is not to identify all likely materials, because the panel did not have the resources to do so. Rather, it was to give the interested reader a brief view of the potential effects of changes in electric propulsion on ship design.
Increased DoD Needs for Electrical Power Generation
Some key reasons that use of electric power is likely to increase in DoD systems are the following:
-
The force as a whole will have fewer uniformed personnel. Eliminating sailor positions will decrease the direct and indirect personnel costs of defending America. However, the tasks to which those people were assigned may not be eliminated, so more automation, robotics, and power must replace them.
-
Components of the DoD will be greener. While a major tenet of green philosophy is conservation, removing pollution from the environ-ment is not without an energy cost. Preprocessing of fuels and post-processing of exhaust will push demand. Reducing a ship’s waste stream or increasing shipboard air quality, for example, will require more electrical power.
-
Information technology (IT) will consume an increasingly large amount of power. For example, aboard-ship IT consists of not only the ship’s computer network, but also the transmitters and receivers to move information on and off the vessel. As signals become more complex, the power needed to generate and process signals must increase.
DoD is moving in the direction of more-electric systems, including electric propulsion. In January 2000 the Secretary of the Navy announced that the next generation of warships would be propelled by an electric drive system.
Advantages of Electric Propulsion in Ship Design
Compared to the standard mechanical drive system that uses reduction gears, there are many advantages to an integrated electric propulsion system:
-
Increased power flexibility allows significant amounts of power to be directed either to the ship’s service power system, to the ship’s propulsion system, or to weaponry, depending on the situation.
-
Increased design flexibility eliminates the reduction gear and the drive train, opening a completely new design space for future ships and allowing large, heavy power-generating equipment to be located anywhere in the ship.
-
Reduced total ownership cost means that power generators can operate at a more efficient speed almost continuously, thus significantly increasing efficiency while reducing fuel cost and engine room staffing.
-
Leveraged commercial electronic and electromagnetic technology replaces reliance on a mechanical drive manufacturing base that no longer produces commercial vessels, with reliance on equipment (e.g., insulated gate bipolar transistors) already available from the commercial power industry.
-
Commonality allows major pieces of electric propulsion equipment to be used in virtually every warship in the U.S. Navy, facilitating modular spare parts usable across platforms.
-
Reduced environmental emissions result from integrating the ship’s service and propulsion power systems. Remaining prime movers can operate at high efficiency, reducing exhaust emissions.
-
Enhanced modularity provides parallel design processes and facilitates modernization and repair on vessels while significantly reducing manufacturing cost.
-
Reduced overall maintenance requirements allow prime movers to operate at their peak performance range at relatively constant power levels for long periods.
-
Increased payload capacity reduces fuel load out, allowing the platform more weapons or ones with greater range, or keeping them longer on station time.
-
Increased automation means that automatic or autonomic systems carry the burden of operating the system, reducing the number of people in the loop.
-
Flexibility/upgradability allows DoD to replace existing combat systems with new ones that use significantly more power without signifi-cantly affecting maximum vessel speed.
DoD-Specific Technology Needs
An array of issues must be addressed before electric propulsion systems become a reality. Many are unique to DoD and will require new approaches to system design and architecture, advanced modeling of electromagnetic characteristics, and, equally important, advances in new materials and processes for making them. Some of the major considerations from the DoD perspective include:
-
Power density. The real estate aboard a military vessel is in high demand, yet DoD is challenged to insert more payload into smaller and cheaper vessels. Because space requirements in today’s commercial market are not as restricted the military cannot leverage on industry.
-
High power levels. One way the marine industry is attempting to mitigate the power density problem is by raising the power levels associated with the propulsion system, but this raises a myriad of issues not previously experienced by marine propulsion engineers. The potential power required of DoD weapon systems only exacerbates the problem. Thermal management becomes an even more critical design criterion and safety of operators and maintenance workers demands more attention.
-
Electromagnetic interference. As automation pervades every area of combat, electromagnetic interference soon becomes critical. Power conditioning equipment is susceptible to the electrical noise generated by the high current and high voltage loads and sources that surround it. Electromagnetic characteristics can also contribute to the ship signature. The commercial industry does not support research in this area, but it is critical to DoD.
-
Shock and impact. This area receives no attention from industry, but it clearly must be addressed for DoD.
Related Materials Issues
A key near-term concern is high-frequency switching and the associated thermal management problems in a power-dense environment. While silicon carbide switching technology is now under development, by 2020 it may be necessary to have available additional options for high-tempera-ture and efficient power-switching and conditioning components. Power electronics were discussed in Chapter 5. Many of the materials technologies already described may help solve these large-scale power problems. For example, increased use of DC power components could eliminate the
need for power conditioning and simplify its distribution. DC promotes the use of components whose raw electrical output is DC, such as fuel cells, and DC has attendant benefits in terms of signature reduction, survivability, and damage control.
The need to carry large amounts of power at low loss while minimizing the volume of power carriers argues for advanced high-temperature superconductors with high critical currents. Similarly, the need for minimizing volume aboard military platforms argues for high-temperature superconducting (HTS) motors, which will have a profound impact on ship architecture, performance, and staffing requirements. These motors should have major advantages over conventional induction motors: It is projected that they will be only 20 percent of the size and 33 percent of the weight of conventional motors. If built in the nearer term, such motors would likely use HTS ceramic superconductors in a metal matrix operating at 77 K (liquid nitrogen). New MgB2 superconductors show considerable promise but at present have a Tc of only 39 K, and would probably run in liquid hydrogen coolant gas. While liquid hydrogen might be acceptable in a civilian application, there are serious concerns about producing and storing significant amounts of it on a warship, where hydrogen’s high explosivity would be a real issue in combat. Nevertheless, as MgB2 and other alloys appear, the Tc may increase to the point where inert liquid gases could provide cooling.
Other materials developments useful for all-electric platforms are advanced dielectric materials for capacitors and high-strength composites for flywheels and compulsators; specific materials developments identified for these components are described elsewhere in the report. The panel did not have the resources to address in depth all the materials issues in this area, but recommends that this be done once a preliminary design for an all-electric platform is completed and device operating parameters are identified. This may result in identifying new materials needs in such categories as high-temperature (1000°F) low-loss electrical insulation; high-performance heat sinks; or EMI shielding materials.
Integrated platform electrical power and propulsion systems will also require improved modeling and analytic tools and advanced algorithms to model effects and interactions among systems and material characteristics in this highly complex environment. Detailed system modeling tools must reflect integrated power-system control and transient response as well as modeling local components. It is vital to understand how electromagnetic parameters scale differently from either hydrodynamic characteristics or
thermal parameters; scaling the electric propulsion system is a challenging task, but there is a shortage of empirical data to support this type of modeling, and the database must be more robust if development costs for full-scale electric propulsion systems are to be contained.
PROJECTED LIGHTWEIGHT ARMOR AREAL DENSITY GOALS
Table D-3 presents projected areal density (AD) goals for lightweight body armor and transparent armor in the period 2000-2030. The main body of this report contains additional information.
DOD RELIANCE ON ENERGY SOURCES
National energy dependence, particularly reliance on energy imports, has been the subject of considerable policy discussion for many years and national energy source and consumption trends continue to be discussed, debated, and addressed at the highest levels in this country because of their implications for national security. While this is not strictly a materials issue, the committee agreed that a brief discussion of total DoD energy usage, dependence on various sources, and energy dependence through 2020 was desirable. This section examines the major energy sources: fossil fuels and nuclear energy.
TABLE D-3 Goals for Future Armor Areal Density
|
Areal Density (lb/ft2) |
|||
Armor Type |
Threat |
Present |
Projected, 2010–2015 |
Projected, 2020–2030 |
Fabric torso protection |
Fragments |
1.38 |
1 |
0.75 |
Rigid fabric helmet |
Fragment |
2.12 |
1.7 |
1.5 |
Rigid fabric/composite torso |
0.30-caliber ball |
6.1 |
3.5-4.5 |
<3.5 |
Torso |
0.30 AP |
6.5-7.5 |
<5 |
<3.5 |
Transparent |
0.30 AP |
23-33 |
<10.5 |
<8 |
|
(glass/polymer) |
(ceramic/polymer) |
(ceramic/polymer) |
|
SOURCE: S. Wax, DARPA, personal communication; J. Ward and P. Cunniff, Soldier Systems Center, Natick, personal communication; Cunniff (1999a,b); and DARPA (1998). |
Dependence on Fossil Fuel
In FY97 total U.S. energy consumption from all sources was 94.21 quads (1 quad = 1 × 1015 British thermal units [BTUs]). For that year, total federal government usage of energy was 1.53 quads. DoD used 1.13 quads in fossil fuel energy, approximately 1.2 percent of the U.S. total (DOE, 1999)—down from 1995, when DoD energy usage was 1.4 percent (1.19 quads) of the U.S. total of 87.3 quads (DOE, 1997).
Of the DoD total for FY97, 58 percent (approx. 0.65 quads) was used for actual support of military operations and training, with the remainder used for buildings and nontactical vehicles. Major energy sources were traditional fuels for military platforms (e.g., jet fuel, diesel) and natural gas and electric power supplied by a variety of land-based systems for fixed installations. No matter how it is calculated, it is clear that DoD energy usage to support the warfighter and military platforms is a very small percentage of total U.S. energy consumption. In addition, during a major crisis national security needs will be given priority, and DoD should have access to whatever energy is needed to assure continuity of its operations. While it is therefore likely that sufficient energy will be available to the DoD in future crises, a question remains about the form in which that energy would be supplied. In particular, will DoD energy consumption patterns change between now and 2020 and, if so, how?
First, 42 percent of DoD’s energy consumption today is supplied to buildings, other fixed assets, and nontactical vehicles. Most of these installations are located within this country, and the energy sources supplying their needs are likely to be those supplying energy to other U.S. infrastructure. The current strong push within the government to reduce energy consumption per square foot by using high-efficiency energy systems and smart technology is expected to continue. At the same time, there is a push to reduce the number of installations by consolidation; this should reduce energy consumption for DoD-owned real property and buildings. Increased efficiency in use, transmission, storage, and production, combined with reduced demand and more efficient systems, offer considerable potential for reduction in this DoD sector.
The other 58 percent of DoD energy consumption is used to power military platforms for operations and for training (the latter being a major contributor to energy consumption, as it is present during peacetime as well as war). There is a continuing push here, too, for enhanced fuel efficiency for many reasons. A recent report of the Defense Science Board
(2001) examined DoD fuel reliance and found that greater fuel efficiency could lead to many benefits, among them enhanced platform stealth, reduced logistics tail, reduced vulnerability of supply lines, and an ability to build up forces faster. As an example, one goal of the Army transformation is to field a future combat system that is lighter weight, more mobile, and more fuel-efficient, because fuel today accounts for 70 percent of the Army tonnage that must be shipped.
There are several ways to reduce DoD fossil energy dependence. More use of alternative energy sources is one. Nuclear and solar power both have potential for certain DoD applications, and geothermal may have some naval applications. Wind and hydropower are unlikely to provide primary power for major platforms. Novel materials can affect many of these modes and may reduce DoD energy consumption through more efficient propulsion technologies; lightweight, high-specific-stiffness and strength structures; vehicle armor approaches that do not rely solely on areal density for protection but use lighter materials and novel protection schemes to reduce weight; higher-energy-density batteries, and fuel cells capable of direct electrochemical conversion; improved fuels; and smart electronics that use energy more effectively. Higher efficiency energy-harvesting materials and devices (e.g., PV conversion) might also augment available energy in specific circumstances.
The full panel report (Chapter 4) discussed the reliance on logistic fuels. It was concluded that both jet fuel and diesel would still be required by 2020, augmented perhaps by methanol, hydrogen (reformed from logistic fuels or otherwise manufactured), and possibly other hydrocarbons. That conclusion is discussed in the fuel cell section of the chapter.
In summary, DoD fossil fuel energy consumption currently represents only a small fraction of total U.S. energy consumption. Because of the systems and infrastructure already in place, it is likely that major energy sources currently used will still be of primary importance in 2020. In particular, fossil fuels will remain crucial to the majority of land and aerospace propulsion applications. While DoD energy sources are likely to reflect those used by society at large, the gradual introduction of new materials, systems, and capabilities and more efficient technology will improve energy usage. In the case of fuel cells, there is likely to be a gradual shift toward alternative fuels by 2020. On the whole, however, it is likely that current fuels (JP-8, diesel, and nuclear) will still make up the bulk of energy sources used by DoD systems in 2020.
Dependence on Nuclear Energy
Within the DoD, the Navy has been the primary user of nuclear power. The decades of nuclear power effectiveness that emerged from the early days of Adm. Rickover’s Nautilus program have been impressive. In 1986 the U.S. Navy was operating or building 6 aircraft carriers, 9 cruisers, 39 ballistic missile submarines, and 97 attack submarines, all with nuclear propulsion plants, without any major incidences or measurable release of contamination.
Removing warship reliance on fossil fuel provides a significant military advantage. America’s military commitments will continue to be worldwide. The ability of our nation’s foremost projectors of power, the nuclear aircraft carriers, to transit to any location in the world at sustained flank speed is currently hindered only by the fact that the support ships that must accompany them are conventionally powered. America’s nuclear-powered vessels are able to remain on station indefinitely, while the fossil-fueled vessels require significant infrastructure for fuel support.
Over the past 30 years, much of the controversy over nuclear power has been focused on civilian nuclear facilities. High-visibility accidents that released radioactivity at Three Mile Island, Chernobyl, and recently in Japan have heightened public concern about the safety, environmental impact, and total cost associated with harnessing nuclear energy. This directly affects DoD strategy for further acquisition of nuclear-powered assets. Indeed, only the unique requirements of aircraft carriers and submarines justify the cost of incorporating nuclear propulsion plants today. However, the current revolution in warship propulsion system design combined with the evolving all-electric warship offer an opportunity for DoD to reevaluate its use of nuclear power as an energy source. Consider the following:
-
Today the world’s energy comes from petroleum (~40 percent), coal (~25 percent), natural gas (~25 percent), hydroelectric (~5 percent), and nuclear (~5 percent).
-
Well over 95 percent of the mobile power generated by DoD platforms (tanks, boats, ships, trucks/autos, aircraft) use petroleum.
-
DoD mobile platforms rely predominantly on fuel oil from sources that the United States does not control.
-
Because even the most efficient fossil fuel engines consume their own weight in fuel within half a day, fuel storage determines overall
-
systems weight, and fuel supply dominates logistics concerns.
-
Over nearly 50 years, nuclear accidents have been few and the DoD track record on nuclear safety has been nearly flawless.
-
Investment in nuclear power R&D is low compared with investment in oil, gas, wind, and photovoltaics, yet it has been estimated that, with sufficient investment, nuclear generation of electricity can be cheaper than generation by a combined-cycle gas plant.
-
DoD’s strategic vision of an all-electric warship/combat vehicle highlights advantages of nuclear generation for (1) warfighting effectiveness, (2) reduced total ownership costs, and (3) increased survivability.
Nuclear electric generators may significantly increase specific power output. A new generation of high-power-density nuclear power plants is possible but would require substantial R&D investment. Feasibility studies of thermonuclear-PV direct energy conversion being conducted at the Knoll’s Atomic Power Laboratory show the clear potential of this technology.
As noted elsewhere in this report, fuel cells are considered a desirable energy source for future defense systems. The continuously renewable hydrogen used by fuel cells of the future could come from nuclear power plants (see the section above on fuel cells). Near-term refinement and deployment of fuel cell power plants, using the existing fossil-fuel delivery infrastructure would make possible long-term use of fuel cell power plants by all facets of DoD. Fusion reactors continue to show great promise. These reactors do not have the dangerous radioactive byproducts of the fission reactor, but they are technologically much more challenging.
Dependence on liquid fossil fuels began in the last half of the 19th century and throughout the 20th mankind has relied on them heavily. However, their price stability and availability cannot be guaranteed in the 21st century. Diversification of DoD energy sources to use more nuclear power is strategically prudent as well as technically and economically justified. Advances in the materials used in electromagnetic machinery, such as high-field permanent magnets and high-temperature superconductors, present opportunities to increase electrical power density and efficiency. Some of the potential for materials developments in these areas is discussed elsewhere in this report. The Energy and Power Panel did not have the resources to fully investigate materials challenges in this area but believes that key DoD platforms are likely to require nuclear energy in 2020 and beyond, and the rationale for increased attention to nuclear power systems and associated materials for DoD platforms is sound.
Technologies linked with advances in nuclear power that require further exploration include:
-
Material technologies for motors and generators, such as superconducting magnets, cryogenic coolers, current collectors, high-field permanent magnets, liquid cooling, and active noise control;
-
Materials with high thermal conductivities that are radioactively inert;
-
Fusion reactor materials (likely to stretch beyond 2020);
-
Materials and concepts for acceptable nuclear waste disposal and storage;
-
Materials for very low harmonic motor controllers;
-
Materials and designs for high-power-density and high-performance solid-state inverters and converters; and
-
Electrical substitutes for systems and components that now rely on fluid transport for energy and actuation.
REFERENCES
Alchavan, J.A. 1998. The Chemistry of Explosives. Cambridge, UK: Royal Society of Chemistry Information Services.
Clelland, I., R. Price, and J. Sarjeant. 2000. Advances in capacitor technology for modern power electronics . Pp. 145-148 in Proceeding of the 24th International Power Modulator Symposium, June, Norfolk, VA. Piscataway, NJ: IEEE.
Cooper, P.W., and S.R. Kurowski. 1996. Introduction to the Technology of Explosives. New York: VCH Publishers.
Cunniff, P.November, 1999a. Dimensionless parameters for optimization of textile-based body armor systems. Proceedings of the 18th International Symposium on Ballistics, San Antonio, TX. Lancaster, PA: Technomic.
Cunniff P. November, 1999b, Assessment of small arms (ball round) body armor systems. Proceedings of the 18th International Symposium on Ballistics, San Antonio, TX. Lancaster, PA: Technomic.
DARPA (Defense Advanced Research Projects Agency). 1998. DARPA/ARO/ARL Transparent Armor Materials Workshop Proceedings, November 16-17, 1998, Annapolis, MD.
Defense Science Board, Task Force on Improving Fuel Efficiency of Weapons Platforms. January 2001. More capable warfighting through reduced fuel burden. Washington, DC: Office of the Under Secretary of Defense for Acquisition, Technology and Logistics. Available online at <www.acq.osd.mil/dsb/fuel.pdf>. Accessed April 21, 2002.
DOE (Department of Energy). 1997. Annual Report to Congress on Federal Government Energy Management and Conservation Programs for Fiscal Year 1995, U.S. Department of Energy.
DOE. 1999. Annual report to Congress on Federal Government Energy Management and Conservation Programs for Fiscal Year 1997, U.S. Department of Energy.
Federoff, B.T. 1960. Encyclopedia of Explosives and Related Items, B.T. Federoff, ed. Dover, NJ: Picatinny Arsenal.
Grätzel, M. 2001. Photoelectrochemical cells. Nature 414(6861):338-344.
Haile, S.M., D.A. Boysen, C.R.I. Chisholm, and R.B. Merle. 2001. Solid acids as fuel cell electrolytes. Nature 410(6831):910.
Hamnett, A., and P. Christensen. 2000. Energy conversion. P. 432 in The New Chemistry, N. Hall, ed. Cambridge, U.K.: Cambridge University Press.
Hibino, T., A. Hashimoto, T. Inoue, J.-I. Tokuna, S.-I. Yoshida, and M. Sano. 2000. A low-operating-temperature solid oxide fuel cell in hydrocarbon-air mixtures. Science 288(5473):2031-2033.
Hirschenhofer, J.H., D.B. Stauffer, R.R. Engelman, and M.G. Klett. 1998. Fuel Cell Handbook, Fourth Edition. Parsons Corp. Available online at <http://www.fuelcells.org/library/FCHandbook.pdf >. Accessed March 3, 2002.
Kornmann, X., L.A. Berglund, J. Sterte, and E.P. Giannelis. 1998. Nanocomposites based on montmorillonite and unsaturated polyester. Polymer Engineering and Science 38(8):1351-1358.
Larmine, J., and D. Andrews. 2000. Fuel Cell Systems Explained. Chichester: John Wiley & Sons.
Messersmith, P.B., and E.P. Giannelis. 1995. Synthesis and barrier properties of poly(e-caprolactone)-layered silicate nanocomposites. Journal of Polymer Science: Part A: Polymer Chemistry 33:1047-1057.
Murray, E.P., T. Tsai, and S.A. Barnett. 1999. A direct-methane fuel cell with a ceria-based anode. Nature 400(6745):649-651.
Nolas, G.S., J. Sharp, and H.J. Goldsmid. 2001. Thermoelectrics: Basic Principles and New Materials Developments. Berlin: Springer-Verlag.
Park, S.D., J.M. Vohs, and R. Gorte. 2000. Direct oxidation of hydrocarbons in a solid-oxide fuel cell. Nature 404(6775):265-267.
Venkatasubramanian, R., E. Siivola, T. Colpitts, and B. O’Quinn. 2001. Thin-film thermoelectric devices with high room-temperature figures of merit. Nature 413(6856):597-602.
Vining, C.B. 2001. Semiconductors are cool. Nature 413(6856):577-578.