2
Introduction to Instrumentation
This chapter introduces the subject of instrumentation in general; defines the particular instrumentation that is the subject of this report, advanced research instrumentation and facilities (ARIF); and gives examples of ARIF used in various fields.
WHAT IS INSTRUMENTATION, AND WHY IS IT IMPORTANT?
Instruments have revolutionized how we look at the world and refined and extended the range of our senses. From the beginnings of the development of the modern scientific method, its emphasis on testable hypotheses required the ability to make quantitative and ever more accurate measurements—for example, of temperature with the thermometer (1593), of cellular structure with the microscope (1595), of the universe with the telescope (1609), and of time itself (to discern longitude at sea) with the marine chronometer (1759). Instruments have been an integral part of our nation’s growth since explorers first set out to map the continent. The establishment of the US Geological Survey had its roots in the exploration of the western United States, and its activities depended critically on advanced surveying instruments.
A large fraction of the differences between 19th century, 20th century, and 21st century science stems directly from the instruments available to explore the world. The scope of research that instrumentation enables has expanded considerably, now encompassing not only the natural (physical and biologic) world but
also many facets of human society and behavior. Instrumentation has often been cited as the pacing factor of research; the productivity of researchers is only as great as the tools they have available to observe, measure, and make sense of nature. As one of the committee’s survey respondents commented,
without continued infrastructure support…. We will see many young investigators changing the nature of the projects and science they do to areas that have less impact but assure better chances of success. The lack of instruments or the ability to upgrade aging local facilities simply dictates the science done in the future.1
Cutting-edge instruments not only enable new discoveries but help to make the production of knowledge more efficient. Many newly developed instruments are important because they enable us to explore phenomena with more precision and speed. The development of instruments maintains a symbiotic relationship with science as a whole; advanced tools enable scientists to answer increasingly complex questions, and new findings in turn enable the development of more powerful, and sometimes novel, instruments.
Instrumentation facilitates interdisciplinary research. Many of the spectacular scientific, engineering, and medical achievements of the last century followed the same simple paradigm of migration from basic to applied science. For example, as the study of basic atomic and molecular physics matured, the instruments developed for those activities were adopted by chemists and applied physicists. That in turn enabled applications in biological, clinical, and environmental science, driven both by universities and by innovative companies. A number of modern tools that are now essential for medical diagnostics, such as magnetic resonance imaging scanners, were originally developed by physicists and chemists for the advancement of basic research.
WHAT IS “ADVANCED RESEARCH INSTRUMENTATION AND FACILITIES”?
Borrowing from the terminology used by Congress in its request, the committee’s study focuses on the issues surrounding a particular category of instruments and collections of instruments, referred to as advanced research instrumentation and facilities. In the charge to the committee, ARIF is defined as instrumentation with capital costs between $2 million and several tens of millions of dollars. In that range, there is no general instrumentation program at either the
National Science Foundation (NSF) or the National Institutes of Health (NIH), yet they are the primary federal agencies that support instrumentation for research outside the national laboratories. The instruments and facilities in this price range fall under neither NSF’s Major Research Instrumentation program nor its Major Research Equipment and Facilities Construction account. The committee found that there are no general ARIF programs at the other federal funding agencies.
The committee identifies other characteristics of ARIF that should be part of its definition. Many qualities distinguish ARIF from more easily acquired instrumentation. The capital cost of ARIF is not its distinguishing factor, and thus many of the characteristics of and challenges associated with ARIF may apply to instruments and facilities costing less than $2 million. ARIF are
-
Difficult for individual investigators to obtain and more commonly acquired by large-scale centers or research programs; it is often necessary to have the consensus of a field when attempting to find support for such an instrument or collection of instruments.
-
Often in need of a substantial institutional commitment for its acquisition, including the availability of proper space and continued upkeep. Multiple federal and nonfederal sources are needed to meet the initial acquisition cost, but the costs of operation and maintenance often require a substantial and long-term financial commitment from the host institution. Many academic institutions—even major research universities—have no ARIF, and institutions that do have ARIF generally have no more than a handful of such instruments or facilities.
-
Often dependent on PhD-level technical research support staff to ensure that researchers are able to take full advantage of the unique capabilities of the instrument and to keep them in proper operating condition.
-
Dependent on relatively high-level decision-making. The investment process for ARIF is likely to include the head of a directorate of NSF, a division or directorate external advisory committee, and the vice-president or vice-provost of research at a university. Identifying or renovating appropriate space for an instrument may require the approval of a university provost or president.
-
Managed by institutions, not individual investigators, because their administration requires a large financial commitment.
-
Often funded in ways that cannot be easily tracked. Acquiring instruments and facilities often requires multiple sources of funding to meet the initial capital cost. As a result, it is difficult to obtain an accurate picture of contributions of institutions, states, federal agencies, and other supporters.
“Advanced research instrumentation provides a technological platform to answer the hardest, unanswered questions in science. An investment on the order of magnitude of 10 or 100 million dollars will pay off many times over if it opens up opportunities to discover new sources of energy, cures for diseases, etc. Beyond potential revenue generating applications, having access to advanced research instrumentation also opens up avenues for fundamental discoveries, the implications of which may be currently unfathomable.” Melissa L. Knothe Tate Associate Professor Case Western Reserve University Response to Committee Survey on Advanced Research Instrumentation |
ARIF includes both commercially available instruments and specially designed and developed instruments and both physical and nonphysical tools. Specially developed instruments are assembled from many less expensive components to make a new, more advanced and powerful instrument. ARIF may be single standalone instruments, networks, computational modeling applications, computer databases, systems of sensors, suites of instruments, and facilities that house ensembles of interrelated instruments. A number of different funding mechanisms support the wide diversity of ARIF.
ARIF can be loosely categorized in two distinct types of use. Some are “workhorse” instruments, essential to everyday research and training. Others are “racehorse” instruments, newly developed or constantly developing, that are perched on the cutting edge of research. Racehorse instruments, because they are novel, are often easier to justify to potential funders. Both workhorse and racehorse instruments are vital for research, and finding the right balance between the two is a challenge.
The “facilities” portion of “ARIF” was incorporated by the committee to emphasize that some research fields and problems require collections of advanced research instrumentation. As has been described earlier, the changing face of research has demanded that a wide array of instruments be brought to bear on a single problem. Collections of instruments are often essential for meaningful research to occur. To solve some types of scientific problems or to engineer new materials, multiple instruments are necessary to carry out a series of steps or processes. Complementary instruments are more effective when housed side by side and may be far more productive than each one is individually.
Historically, centralized facilities have played a large role in research involving
ARIF instrumentation by consolidating resources, increasing collaboration, and making available rare or unique resources to a large number of users. Most publicly funded centralized facilities are located at universities and national laboratories. The state of US research facilities is often cited as an indicator of the nation’s long-term international competitiveness in research. For example, a 2000 National Academies Committee on Science, Engineering, and Public Policy study of materials facilities noted that “rapid advances in design and capabilities of instrumentation can create obsolescence in 5–8 years.” The study further noted that the overall quality of characterization services provided by materials facilities supporting universities and industry had “lost substantial ground” to Japan and Europe.2 The committee distinguishes between facilities and centers. A center is defined as a collection of investigators with a particular research focus. A facility is defined as a collection of equipment, instrumentation, technical support personnel, and physical resources that enables investigators to perform research.
In referring to ARIF, the committee excludes facilities that house large assemblies of unrelated or only loosely related equipment and that generally require no targeted support staff. Different research fields require different types of ARIF, and some fields have a larger demand for ARIF than others. ARIF are not distinguished by the diversity of research fields or geographic regions it supports.
EXAMPLES OF ARIF
The research community recognizes the importance of instruments. The National Science Board (NSB) recently identified eight Nobel prizes in physics that were awarded for the development of new or enhanced instrumentation technologies, including electron and scanning tunneling microscopes, laser and neutron spectroscopy, particle detectors, and the integrated circuit.3 Nobel prizes for the development of instrumentation have been awarded in chemistry and medicine for instrumentation related to nuclear magnetic resonance (NMR) or magnetic resonance imaging, and many were also awarded at least in part for the development of ARIF. Many of the ground-breaking instruments that qualified for a Nobel prize or contributed to Nobel prize-winning work began as ARIF and through development have become widely available and more affordable.
Table 2-1 lists the types of ARIF reported to the committee in its survey of institutions. The results of this survey are known to be incomplete and not repre-
TABLE 2-1 Examples of ARIF, by Field
Field |
Selected Instruments or Facilities |
Capital Cost (millions of $) |
Astronomy |
Telescope, spectrograph, infrared camera for Magellan |
3.9-5 |
Biology |
Proteomics-protein structure laboratory |
2.7 |
Cyberinfrastructure |
Supercomputer |
2.0-5.0 |
Geosciences |
Ion microprobe, earthquake sensor testing laboratory |
2.8-3.8 |
Materials |
Electron-beam lithography system, semiconductor production system |
2.3-2.5 |
Human and animal imaging |
Magnetic resonance imager, human and animal |
2.2-2.8 |
Spectrometry (NMR) |
NMR spectrometer, 800-900 MHz |
2.2-4.8 |
Physics |
Infrared camera, pulsed electron accelerator |
2.0-15.0 |
Space |
MegaSIMS (isotope analysis) |
3.5 |
Facility-supporting equipment |
Helium refrigerator supplying helium for superconducting magnets at the National Superconducting Cyclotron Laboratory |
2.9 |
Source: Committee on Advanced Research Instrumentation survey of academic institutions, 2005. |
sentative of the state of ARIF on university campuses. Notably absent from this table, for example, are cybertools other than supercomputers, which cost much to develop but little to use. Computer modeling programs are used often by the chemistry and biology community. The cost of acquiring these computational modeling programs is often negligibly small, but the cost of creating them is often substantial. The computational chemistry program, NWChem, for example, cost around $10 million to create and is distributed free. Further details about the committee’s survey and the ARIF reported in it can be found in Appendix C. Table 2-1 is followed by descriptions of several types of ARIF.
Imaging Technologies: From Physics to Biology
Imaging technologies provide many of the best-known examples of the evolution of modern instrumentation. Fifty years ago, studies of the effects of magnetic fields on the nuclear spin states of molecules were at the forefront of esoteric physics research. The earliest magnetic resonance spectrometers were inexpensive to build (they could literally be cobbled together by graduate students from spare radar parts); this was fortunate because measuring nuclear spin properties had no conceivable application. If sensitivity and instrument performance had stayed the same, NMR still would have no conceivable application.
Instead, today magnetic resonance is a fundamental technique for biological imaging and the most important spectroscopic method for chemists, the only one that measures the structure of proteins in their natural environment (in solution). Since 1980, the sensitivity of the best commercially available NMR spectrometers
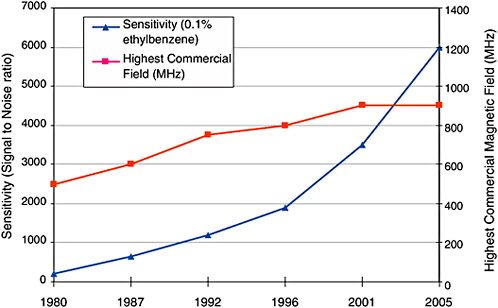
FIGURE 2-1 Historical capability of NMR spectrometers.
Source: Razvan Teodorescu, “Bruker Biospin Magnets.” Presentation to the National Research Council Committee on High Magnetic Field Science, December 8, 2003.
has improved by a factor of 30. With that advance alone, NMR spectra could be acquired 900 times faster today than 25 years ago. Improvements in resolution and pulse sequences make the advances in NMR spectrometry even more dramatic. Figure 2-1 shows how NMR resolution and sensitivity have progressed since 1980.
Modern, very-high-field NMR spectrometers (high fields help to resolve the many atoms found in large molecules) are complex instruments; the most advanced machines today cost millions of dollars. The next generation, which pushes to still higher magnetic field strengths, will require a concerted effort in superconductor physics and radiofrequency design but will create even further dramatic extensions of the applicability of the technique.4
The pioneers of magnetic resonance would never have dreamed that 50 years later the International Society of Magnetic Resonance in Medicine would have 2,800 papers and 4,500 attendees at its annual meetings. Today, magnetic resonance imaging is a mainstream diagnostic tool, and functional magnetic resonance
imaging (literally, watching people think) promises to revolutionize neuroscience and neurology. Again, the applications and the expense are intimately coupled to the sophistication of the technology: a modern, commercially available 4 Tesla whole-body magnetic resonance imager can easily cost $10 million to acquire and site, and it requires highly specialized technical staffing to maintain its perfor-
mance. For the institutions that responded to the committee’s survey, the annual cost of operation for magnetic resonance imagers averaged 10% of the capital cost.
Modern methods in optical and x-ray imaging also reflect the evolution from physics to more applied science. They are not simply descendants of van Leeuwenhoek’s crude microscope and Röntgen’s x-ray hand picture; they embody
and enhance our understanding of molecular and cellular structure and function. It is only a slight exaggeration to say that the most important application of the crude lasers of the 1960s was as inspiration for science-fiction television and movie weapons. Today, advanced laser systems permit microscopy hundreds of times deeper into tissue than would be possible with an ordinary microscope, and they are central to the rapidly growing field of molecular imaging.
The National Cancer Institute has identified molecular imaging as an “extraordinary opportunity” with high scientific priority for cancer research. The most promising approach is the development of new technologies and methods to improve the imaging and molecular-level characterization of biologic systems.
In the committee’s surveys of researchers and institutions, NMR spectrometers were among the most commonly cited individual instruments, and advanced models were among the most commonly sought. The availability of ARIF in general was of concern to many researchers for whom access to increasingly advanced instruments was the key to advancing science and providing solutions to societal problems. The NMR spectrometer, as it becomes more and more sophisticated, exemplifies the issue. As one researcher noted,
there is an increasing need for advanced research instrumentation in many fields. Many instruments that start out appearing to be expensive and esoteric rapidly become mainstream. The good side of this is that these instruments fuel impressive scientific results. The bad side is that scientists who do not have access to these instruments tend to fall behind in terms of their results and in what experiments they can propose in grant applications.
… Five or six years ago, few labs had access to very high field spectrometers (750 MHz or above), but now the field has been pushed ahead to where many … projects require such instrumentation. A significant number of researchers have access to these machines, but many either don’t have access or must drive/fly long distances to obtain access. While on paper it sounds fine to ask a researcher to travel to a high field spectrometer, in practice this is very cumbersome and does not lead to cutting edge results. For any particular NMR project, a dozen or more different NMR experiments must be carried out on a sample…. Traveling back and forth to a “richer” or better endowed university is not conducive to getting results.5
High-Speed Sequencers and the Human Genome Project
One of the major accomplishments of science in the 20th century was the deciphering of the human genome. That achievement made it possible to understand the molecular basis of human life in unprecedented detail. The potential for
improving health and curing disease has already been demonstrated, but most of the benefits remain to be seen. The achievement will provide the basis of discoveries far into the future.
The genetic information in DNA is stored as a sequence of bases, and DNA sequencing is the determination of the exact order of the base pairs in a segment of DNA. Two groups, in the United States and the United Kingdom, first accomplished sequencing in 1977 and were awarded the 1980 Nobel prize in chemistry. However, their approaches were time-consuming and labor-intensive. Further
advances came in 1986-1987 with the development of fluorescence-based detection of the bases. That led quickly to automated high-throughput DNA sequencers that were soon commercialized and made generally available to the research community. However, the speed of those devices was still not sufficient to decode the human genome in any reasonable amount of time.
Beginning in 1990, the pressures of approaching the daunting task of sequencing the human genome produced a number of new advances, which resulted in a fully automated high-throughput parallel-processing device that was 10 times faster than the older method. The progress and success of the Human Genome Project constitute a case study in instrumentation and of how, without develop-
ment, it can become a pacing factor for research. The leaders of the sequencing efforts at the Department of Energy Office of Science and NIH recognized that the existing technology was not capable of sequencing fast and cost-effectively. As a result, they invested substantially not only in researchers but in the further development of sequencing technologies. The tandem approach proved very successful.
Genome sequencing requires the assembly of millions of fragments into a complete sequence. By itself, the mechanical process of sequencing was not sufficient to map the human genome in a reasonable time. The project was aided by computer algorithms developed by researchers in the late eighties and early nineties. The confluence of hardware and software development made it possible to complete the human genome sequence years before it had been considered possible. It also provided a general approach to large-scale sequencing that has resulted in the understanding of the genomes of a wide variety of organisms. The knowledge of genomes of a number of organisms has vastly accelerated discovery in basic biology research.
The history of the genome project shows how technology development can influence the course of discovery. Hardware and software were both needed and
were synergistic. The parallel development of sequencers and software demonstrates that not only key insights but also incremental improvements can make a qualitative difference in the progress of science.
Cybertools
Today, computers are vital tools to scientists and engineers. Indispensable for communication and often used in conjunction with many instruments, the computer can also be a scientific instrument itself. This section gives examples of three types of cybertools that are fundamental to several fields of research: software, data collections, and surveys.
Although one of the first scientific applications of digital computers in the 1940s was to try to predict the weather—with grants from the US Weather Service, the Navy, and the Air Force to John von Neumann at the Institute for Advanced Study at Princeton University—scientific applications software aimed at obtaining
Gaussian and the Nobel Prize In 1998, John A. Pople was awarded the Nobel prize in chemistry (his colaureate was Walter Kohn of the University of California, Santa Barbara). The citation for Professor Pople refered to “his development of computational methods in quantum chemistry.” The research “tool” that Professor Pople developed was the Gaussian program, which is now one of the most widely used tools in chemical research. The first version of Gaussian was released in 1970 and provided only limited ways of modeling molecular structure and processes. The last release of Gaussian, in 2003, is so advanced it can predict the structure and properties of many molecules to an accuracy that enables chemists to gain a better understanding of the problems they are investigating. |
a better understanding of physical phenomena did not become widely available until the 1960s and 1970s. Scientists and engineers have since developed a broad array of scientific software applications that are acknowledged to be indispensable in the scientist’s toolkit—the software equivalents of the NMR spectrometer and other instruments described above. Examples of such applications software in use today are Gaussian, a molecular modeling code that is used by experimental and theoretical chemists to understand molecular structure and processes better and more easily by performing computer “experiments” rather than chemistry experiments; the Community Climate System Model, which is used by the climate research community to understand the evolution of past and future climates; and CHARMM and AMBER, used by the biomolecular community to understand the structure and dynamics of proteins and enzymes.
Scientific applications, such as Gaussian, often began as small research projects in the laboratory of a single investigator. However, as the capabilities of computers increased, the applications included models of the physical and chemical processes of higher and higher fidelity, and the software became more and more complex. Today, many of the scientific applications involve hundreds of thousands to millions of lines of code, took hundreds of person-years to design and build, and require substantial continuing support to maintain, port to new computers, and continue to evolve the capabilities of the software as new knowledge accumulates. The cost of developing major new scientific and engineering applications can be more than $10 million, and the cost of continuing maintenance, support, and evolution exceeds $2 million per year. Thus, these software applications are well within the category of advanced research instrumentation being considered in this report.
Digital data collections also provide a fundamentally new approach to research. By gathering data generated in studies on related topics, digital data collections themselves become a new source of knowledge. One of the best examples of a large scientific database that is integral to progress in science and engineering is GenBank, the genetic sequence database maintained for the biomedical research community by NIH. GenBank was born at Los Alamos National Laboratory in 1982, well before the beginning of the Human Genome Project. When the Human Genome Project came into being and the number of available sequences exploded, GenBank became an indispensable repository for the data being generated. Today GenBank contains over 49 billion nucleotide bases in over 45 million sequence records, and the amount of data is increasing exponentially with a doubling time
of less than two years. All sequencing data produced by the Human Genome Project must be deposited in GenBank before it can be published in the literature. Because of the unique role now being played by digital data collections in research and education, the NSB recently drafted a report on the subject, finding that such collections are used in most fields of science, from astronomy (as in the Sloan Digital Sky Survey) to biology (as in The Arabidopsis Information Resource).6 It concluded that “digital data collections serve as an instrument for performing analysis with an accuracy that was not possible previously or, by combining information in new ways, from a perspective that was previously inaccessible.” The collections are often fundamental tools of the social sciences, housing extensive survey and census results and archived media.
Especially in the social sciences, the survey itself is a scientific instrument that can cost millions of dollars a year to maintain. Longitudinal surveys, large and often decades-long surveys, are analogs to the telescopes and microscopes of the other sciences. These surveys are not created by single investigators; they are often sources of basic data used by arrays of disciplines. Increasingly, surveys collect not only social data but also biomedical data (e.g., cheek swabs for DNA analysis) or are integrated with satellite down feeds or inputs from air quality sensors. The data from these surveys is expensive to collect and document as well as make publicly accessible to researchers while preserving anonymity and confidentiality. They are very expensive to collect in any given round let alone over time. They are also expensive to document and make accessible as public use files, preserving anonymity and confidentiality. Frequently, the data for such surveys can only be collected by one or two survey research centers in the country, such as the Institute for Social Research at the University of Michigan, that possess the ongoing human and local infrastructure to manage them at affordable scale.
Computational technology has advanced to the point where computers can be used as tools not only for remotely accessing databases and collaborating with other researchers but for remotely accessing and controlling scientific instruments. A 900-MHz virtual NMR facility at the Pacific Northwest National Laboratory, for example, supports a national community of users, roughly half of whom use the instrument remotely. The technology can improve and provide less expensive access to instruments for geographically remote users and can permit more effective use of instruments. The openness of this technology and the ability to book at all hours may be a means to generate more revenue from user fees and thus recoup the facility’s operation and maintenance expeditures.
Distributed Advanced Research Instrumentation Systems
Not all advanced research instrumentation is housed in laboratories. Progress in the physics underlying the technological development of modern scientific instruments and their associated cybertools has given rise to an unprecedented explosion in the scope of basic research in the geosciences and biosciences that relies on field observations. Atmospheric scientists, oceanographers, geophysicists, and ecologists are now tackling and solving fundamental problems that require analysis of large numbers of observations that are both time- and space-dependent. Some of the sensors and tools required to make the necessary measurements can be deployed on familiar mobile instrumental platforms, such as oceanographic ships, research aircraft, and earth-orbiting satellites; but many need to be distributed in sensor networks of local, regional, or even global scale. Both physical and wireless networks can be used to transmit data to off-site storage facilities.
A good example of a distributed sensor network is the Global Seismographic Network (GSN), which consists of 130 seismic stations distributed on continental landmasses, oceanic islands, and the ocean bottom. GSN recording and nearly real-time distribution of seismic-wave parameters measurements at numerous sites over the globe serve the needs of basic research in geophysics (such as seismic tomography of the earth’s interior structure) and of applied geosciences (such as earthquake and tsunami monitoring and seismic monitoring of nuclear testing).
Seismometers were originally developed to study earthquakes, but their modern versions, deployed in geographically distributed networks, record data that can be processed with sophisticated computing methods to produce images of the solid earth. The resulting “seismic imaging” is science’s most important source of knowledge about the structure of the earth’s interior and its consequences for humanity with respect to, for example, mineral and energy resources, earthquakes and volcanic hazards.
Today’s seismograph system takes advantage of modern off-the-shelf hardware for many of its components. Global positioning system (GPS) receivers provide the accurate timing required, off-the-shelf electronic amplifiers generate little noise or distortion, and commercial analog-to-digital converters with true 24-bit or higher resolution are an improvement over custom-designed “gain-ranging” systems; but the primary sensor of a modern seismometer still requires unique design to meet a combination of stringent requirements. To detect the smallest signals above the earth’s background “hum,” the self-excitation of the pendulum sensor by Brownian noise must be less than that caused by shaking the instrument’s foundation with an acceleration of 1 nm/s2 across a wide frequency band of 10−4-100 Hz. Furthermore, to make faithful records of the largest earthquakes, the response across the same frequency band must be linear up to excitation amplitudes that are 1012 times greater than the smallest detectable signals. No company in the United States produces sensors with those capabilities, and no US universities train engineers in seismometer design.
Environmental sensor systems must often be installed in remote field locations, and this poses difficulties not encountered in housing instruments in a laboratory setting. For example, seismometers must be installed in ways that isolate them from drafts, temperature changes, and ambient noise and that protect them from damage by animals and vandalism. Low-power, rugged, and high-capacity data-storage systems are required for remote locations where energy must be provided by fuel cells or batteries recharged by solar or wind-based systems. In locations where Internet service is not available, transmission of data must take advantage of satellites or other telemetry technologies that can substantially add to costs. Although data transmission and interrogation of routine instrument functions can be dealt with remotely, periodic maintenance by technicians is needed
and can account for a large fraction of operation and maintenance costs, especially for large networks with worldwide distribution of stations. Although the combined equipment, installation, and a year of operation and maintenance of an individual station typically will cost between $100,000 and several hundred thousand dollars depending on instrumentation specifications and location (polar regions and ocean-bottom locations are obvious examples of expensive locations), it is clear to the committee that distributed network “instruments” fall well within its definition of ARIF.
Tools for Integrated Circuits
An advanced research instrument may consist of a suite of tools that must be combined to advance a particular field of science and technology and eventually affect society. An excellent example is the microelectronic processing technology
that has been developed over the last 50 years to create integrated circuits (ICs). ICs are found in every electronic product purchased by Americans to enhance our day-to-day living. Communication, education, transportation, defense, health care, and recreation, to name a few examples, have been dramatically transformed by the creation of ICs. In 1947, the first point-contact transistor was demonstrated; it consisted of a sizable chunk of germanium with two gold wires to conduct electricity and enable the demonstration of power gain. A few years later, in 1956, Bardeen, Brattain, and Shockley were awarded the Nobel prize in physics for the discovery of the transistor. Throughout the 1950s and 1960s key technological breakthroughs in crystal growth, ion implantation, photolithography, and planar processing paved the way for the creation of the IC. By fabricating the transistor in a planar form, engineers and scientists could envision methods to interconnect the transistors and begin combining them to perform an unlimited number of functions.
In 1971, Intel introduced its 4004 processor that contained 2,300 integrated and interconnected transistors in a 4 × 5 mm area—about 10,000 transistors/cm2. In 1997, the Intel Pentium II processor contained 7.5 million transistors in an area of about 8 × 8 mm. The Pentium III has 28 million transistors; by 2006, the industry projects a logic transistor density of 40-80 million per square centimeter! In 2000, Kilby was awarded the Nobel prize for the invention of the IC. Today, the microelectronic processing technology industry has sales of over $150 billion per year. To create such amazing ultra-high-density, small-area ICs requires a suite of planar processing tools, each of which can be categorized as an advanced research instrument but all of which are needed to build the IC.
As the capability of an instrument increases, its price also increases. For example, in 1982, a physical vapor-deposition tool cost about $400,000; whereas in 2002 a physical vapor-deposition tool cost $7 million. This rise in cost reflects substantial gains in the precision and repeatability of vapor deposition. Similar increases in equipment costs have occurred for other tools in the suite as resolution has increased, feature size has been reduced, and overlay accuracy has been improved. The incredible gains demonstrated by the microelectronic processing technology industry were facilitated by placing the suite of tools within specially designed space or clean rooms to ensure defect-free high-density ICs; such special space adds further to the cost of ownership of these advanced research instruments.
Nanotechnology
Nanotechnology is a broad field that has stemmed from our recently developed ability to manipulate atomic and molecular objects with dimensions 1-100 nm—a length scale that has become increasingly important in pushing the boundaries of operation and performance. It involves the ability to engineer materials on a
nanometer scale by placing atoms into predetermined locations. In light of the broad goals and possible applications of nanotechnology, a large array of synergistic tools has been developed.
A primary need in nanotechnology is the ability to “see” the locations of specific atoms. That has been accomplished largely through the development of scanning probe microscopes. These types of microscopes have the ability to scan or map a surface line by line at atomic resolution. Scanning probe microscopes are distinct from most other microscopes in using mechanical devices, instead of light and lenses, to image surfaces.
The development of scanning probe technology began in 1981 with the scanning tunneling microscope in Zurich. Scanning tunneling microscopes use quantum principles not only to visualize surfaces but also to manipulate them by, for example, initiating chemical reactions. In 1986, the Nobel prize in physics was awarded to the discoverers of this microscopy technique; the remarkably short
time after the initial discovery indicates its importance. Since then, an array of other scanning microcopies have been developed, most notably the atomic-force microscope in 1986 that measured attractive or repulsive forces between a very fine tip and a sample. That approach allowed the imaging of nonconductive surfaces, which the tunneling technique could not do. As is often the case, sophisticated software is required to make sense of the information generated by these tools and to integrate it into a comprehensible image.
The effects of nanotechnology are beginning to be felt, for example, in materials science (nanotubes and nanoparticles), in the development of smaller computer chips, and in the manipulation of biologic components to create nanomotors. Recently, there has been interest in the interaction of “hard” and “soft” materials, which require special facilities where semiconductor fabrication and biomolecular assembly can take place in concert.
Tools for Space Exploration
The exploration of space and the solar system depends on a number of ground-based and space instruments, such as the Supernova Acceleration Probe, a satellite observatory that probes the history of the expansion of the universe over the last 10 billion years. The exploration of space has also become increasingly dependent on diagnostic equipment, whose focus is characterization of samples that have been returned to the earth from space. Such instruments as the MegaSIMS combination spectrometer (see Table 2-1), which was specially designed to analyze GENESIS NASA solar wind samples, are ARIF that bridge space and earth exploration and enable us to understand better the materials that make up the world around us and the processes that govern its development.
FINDINGS
F2-1: Instrumentation is a major pacing factor for research; the productivity of researchers is only as great as the tools they have available to observe, measure, and make sense of nature. Workhorse instruments, once cutting-edge, now enable scientists to perform routine experiments and procedures much faster. Those tools now have efficiency and sensitivity greater by several orders of magnitude than a decade ago. Research that previously took years to conduct now takes hours. Most of the research funded by the federal government today would be impossible with tools that were developed in decades past.
F2-2: As new research questions are answered, more advanced instrumentation needs to be developed to respond to researcher needs. As a result, the useful life of instruments has shortened dramatically in recent decades, and the demand for new instruments has increased. Instruments that used to be relevant to cutting-edge research for a decade or more today last less than half that time. Older instruments are still useful, but the demand for new instruments is greater and greater.
F2-3: ARIF include both workhorse instruments that are used every day by researchers and racehorse instruments that represent the state of the art or are still developing.
F2-4: ARIF often depend on PhD-level technical research support staff for its proper operation and maintenance and to facilitate use by researchers. ARIF require highly specialized knowledge and training for proper operation and use. Nonspecialists increasingly need ARIF for their research and require dedicated personnel to provide expertise.
RECOMMENDATIONS
R2-1: The committee recommends the following definition of ARIF:
Advanced research instrumentation and facilities (ARIF) are instrumentation and facilities housing collections of closely related or interacting instruments used for research and includes networks of sensors, data collections, and cyberinfrastructure. ARIF are distinguished from other types of instrumentation by being more commonly acquired by large-scale centers or research programs rather than individual investigators. The acquisition of ARIF by scientists often requires a substantial institutional commitment, depends on high-level decision-making at institutions and federal agencies, and is often managed by institutions. Furthermore, the advanced nature of ARIF often requires expert PhD-level technical research support staff for its operation and maintenance.
R2-2: Continued and vigorous federal investment in ARIF is essential to enable cutting-edge research in the future.