3
Pushing the Boundaries of Life
Following its strategic plan, the committee examined the wealth of information concerning the life that we know on Earth, especially in what (from a human perspective) are extreme environments.
3.1
THE LIMITS OF EARTH LIFE
The search for extraterrestrial life is intimately linked with current understanding of Earth life. That is not to say that only Earth-like life could exist on other planets and moons, but it is important to know the limits of environmental conditions that can support the growth of Earth life as a first-order set of criteria for the identification of potential extraterrestrial habitats. The limits of life on Earth may help to define the limits of life elsewhere even though different life forms may have different biochemistries and different origins. In addition, Earth life is possible outside the bounds of extreme conditions found on Earth (for example, the bacterium Deinococcus radiodurans can tolerate levels of radiation not found naturally on present-day Earth, and Escherichia coli apparently can tolerate hydrostatic pressures greater than 10 times the pressures in the deepest ocean trenches1,2). Because we know about only one kind of life, it is logical to look first for life elsewhere that resembles Earth life. Life as we know it requires liquid water, either light or a chemical energy source, and nutrients, including nitrogen, phosphorus, sulfur, iron, and many elements in trace concentration; 70 elements are either required by or interacted with by Earth life.3 Thus, the current search for extraterrestrial life is focused on planets and moons that have or have had liquid water, that have a history of geological and geophysical properties that favor the synthesis of organic compounds and their polymerization, and that provide the energy sources and nutrients needed to sustain life.
Once life appears on a planet, regardless of the circumstances under which it appeared, evolution will push toward occupying every conceivable niche, even when the environments in some niches are very different from that in which life first arose.
In using Earth life as a point of comparison, we are limited by what we know about it. Two issues are being addressed by the astrobiology community: our incomplete understanding of the physiological diversity of Earth life, and the near absence of data on possible alternative forms of carbon-based biochemistries. First, it is clear that little or nothing is known about the physiological diversity of most microorganisms in most Earth environments. For example, examination of the physiological characteristics of some of the organisms in the “unknown majority” has provided new insights into their adaptations to “extreme” environments, those at the fringes of habitability as we know it. A novel marine photosynthetic microorganism that was isolated from deep-sea hydrothermal vents
might be using the black-body radiation from hot sulfides for photosynthesis.4 Newly isolated microorganisms have extended the upper temperature for growth to 121°C and extended the pH limit to below 0. One hyperthermophilic microorganism lacks consensus sequences (nucleotide base sequences in the 16S rRNA gene that are universal in known organisms) in its 16S rRNA and, incidentally, is parasitic on another archaean species.5
To address the second issue, researchers begin by assuming carbon-based life. The key arguments for carbon-based life are the ubiquity of organic (carbon-containing) compounds in the universe and the ability of carbon to form stable compounds with many elements, thus creating the wide variety of structural, catalytic, and informational macromolecules (very large molecules, such as DNA and proteins) that make up Earth life. But how versatile and adequate is the carbon-based life model for environmental conditions that either have not been adequately explored on Earth or extend beyond the bounds found on Earth? Are there alternative carbon-based biochemistries that would allow organisms to exist under more extreme conditions than Earth life can? Embedded in that question are two others: What are the limits of evolutionary innovations in carbon-based life? How do environmental characteristics or extrinsic factors, such as hydrostatic pressure and solute concentrations, affect the limits?
Powerful new molecular methods allow the construction of novel enzymes and, eventually, novel organisms. A viral genome has already been constructed from synthetic oligonucleotides.6 Those “directed evolution” methods have the potential to explore whether it is possible to construct life with novel physiologies, including new metabolic pathways that exploit energy sources not used by extant Earth organisms—such energy sources as ultraviolet radiation, gravity, and thermal gradients. Furthermore, exploration of deep subsurface environments has uncovered microbial communities potentially decoupled from photosynthetic reactions that occur at Earth’s surface. Those communities use magmatic degassing, radiolysis of water, or serpentinization reactions (involving the aqueous alteration of mantle material) to drive their metabolism. The ecosystems persist in environments that have remained essentially unchanged for millions or billions of years and that overlap with conditions favorable for abiotic organic synthesis. Do organisms in those ecosystems contain relict biochemistries that have eluded evolutionary pressures?
Thus, two questions are associated with understanding the limits of carbon-based life: What are the limits of extant Earth life? What are the limits of carbon-based life? With respect to the second question, one of the implications of using Earth-based life as a point of comparison is the need to understand the full range of habitat conditions that can support carbon-based life, including conditions not found on Earth. The tendency is to look for Earth organisms that might be best suited to live under the extreme conditions found on other planets, but that assumes that an extraterrestrial organism would have the same physiological characteristics of an Earth organism if the environmental conditions were the same in the two places. In thinking that way, we assume that life originated in the other place and then evolved physiologies to take advantage of the available habitat conditions. But any planet or moon that has or has had environmental conditions that could support an Earth-like organism might never have had the conditions necessary for a separate origin of life and could be sterile. That may be particularly true for icy planets with liquid water even if they have the cache of chemicals necessary to support life. Could life originate de novo, or would it have to be seeded from a neighboring planet or moon that during its early history had more suitable conditions for spawning life? How many types of environments can lead to the origin of life?
In the case of Earth, various models provide clues to geophysical conditions that may have favored early organic and biochemical stages leading to life. Some models rely on subsurface hydrothermal settings because they can provide all the chemical precursors and catalysts essential to generating complex carbon chemistry. Other models exploit alternative settings, such as meteoritic input, reduced atmospheres, or freshwater ponds. The origin of life is likely to involve multiple environmental conditions that span spatial and temporal dimensions. However, we cannot answer the general question of whether there could be multiple settings for creating different carbon-based life forms. Would a separate origin of life under conditions different from the ones that produced life on Earth create a carbon-based life form capable of different evolutionary innovations, or do rules of organic chemistry limit carbon-based life to the physiological diversity represented by extant Earth life?
Earth may be just one of many models of planets that can evolve complex life. We do not know whether it is even practical or logical to assume that planets that exist outside our perception of a habitable zone could harbor life, particularly life that we know nothing about. Our practical search for extraterrestrial life is focused on water-rich planets and moons because of the possibility that they can support Earth-like life. That does not preclude
other strategies for carbon-based life to thrive in nonaqueous solvents, such as exist on Titan or in the hot sulfuric acid atmosphere of Venus. Even more radical is the possibility of seriously “weird life” on extremely hot, cold, or gaseous giant planets.7,8 We know about only one kind of life, and it is carbon-based, requires liquid water, can evolve, and, given “optimal” environmental conditions, can evolve into complex organisms.
3.2
EXTREMOPHILES AND THE LIMITS OF LIFE
Extreme conditions that limit growth or prove lethal to most organisms can be ideal habitats for others. Extremes of high temperature, high and low pH, high salt concentration, concentrations of toxic metals and organic compounds, and high levels of radiation kill the overwhelming majority of Earth’s organisms. However, organisms in all three domains of life have adapted to many terrestrial extremes. High-temperature, low-pH, and high-salinity environments are probably ancient, as may be frozen environments. Those extreme environments are not rare: most of the ocean is cold and deep, and a vast portion of the subsurface is hot.
There are few natural environments on Earth where life is absent—life is the rule rather than the exception. Microbial life on Earth has proliferated in habitats that span nearly every imaginable physicochemical variable. Until recently, only the very highest temperatures or lowest water activities (desiccation) were thought to render terrestrial environments unsuitable for growth. Now there is evidence that environments that have MgCl2 at concentrations greater than 2.3 M, such as a high-brine lake on the Mediterranean Sea floor, may inhibit life and that this inhibition is due to the ability of MgCl2 to denature biological macromolecules.9 However, such conditions do not necessarily render the environments sterile; many organisms have adapted mechanisms for long-term survival at temperatures more than 100°C above their maximal growth temperature or in a desiccated state. Few of the supposedly sterile environments are actually free of surviving life. Viable microorganisms have been detected, albeit in low numbers, in Chile’s Atacama Desert, perhaps the driest environment on Earth and thought to be an analogue of sterile Martian soil.10 In contrast, although they are rare, some environments with liquid water do not appear to support life; they include water over 400°C at submarine hydrothermal vents that is kept liquid by hydrostatic pressure11 and the high-brine liquid water found in sea-ice inclusions at −30°C. Even in those extreme cases, there is evidence of viable microorganisms that apparently survive exposure to temperature extremes well outside their growth range.12
Several recent review articles discuss the limits of life, the characteristics of extremophiles, and implications for astrobiology.13-15 Most discussions of the limits of life focus on extremes of single physical or chemical conditions, such as temperature, salinity, heavy-metal concentrations, desiccation, and pH. There are also many excellent reviews of single classes of extremophiles that should be consulted for detailed information on their ecology, physiology, and biochemistry.16-20
Individual organisms are often highlighted for their ability to lead the pack in tolerance of or ability to grow under extreme conditions (Table 3.1). In some cases—such as high pH, high hydrostatic pressure, and high metal content—the stated limits for life merely reflect the limits found in natural environments. There appears to be no absolute maximal temperature or minimal concentration of water that will prevent cellular growth. Two distinct classes of extreme environmental conditions are based on how they affect cells. In one, the effects of extremes in pressure and temperature extend into the cytoplasm, and intracellular biosynthesis, metabolism, and macromolecular structures are adapted to function under such conditions. In the other, organisms capable of growing in extremes of pH, salinity, and irradiation and in the presence of high concentrations of organic solvents and toxic metals are adapted either to maintain intracellular conditions that are typical for nonextremophiles or compensate for the extreme conditions. There are some exceptions. A recently described acidophilic archaean, Picrophilus torridus, grows optimally at a pH of 0.7 and apparently maintains an intracellular pH of 4.6.21 Most acidophiles maintain an internal pH near neutrality, and P. torridus must have novel intrinsic factors for stabilizing proteins and nucleic acids at low pH. The extremely halophilic archaeans have an absolute requirement for salt and grow best at salt concentrations of 3.5-4.5 M but can also grow in saturated NaCl (5.2 M). The intracellular functional and structural components of haloarchaeans are adapted to high salt concentration (up to 5 M, mainly KCl), and their enzymes require high salt to maintain their active structure. Other moderately halophilic bacteria grow over
TABLE 3.1 Examples of Bacteria, Archaeans, and Eukaryotes That Grow in Extreme Environmental Conditions on Earth
|
Extreme Value for Growth or Tolerance |
|
|
Condition |
Bacteria and Archaeans |
Eukaryotes |
Examples of Environments |
High temperature |
121°C, Strain 121; 113°C, Pyrolobus fumarii |
~60°C algae, e.g., Cyanidium caldarium and some fungal species |
Submarine hydrothermal systems; geothermal hot springs (e.g., Yellowstone) |
Low temperature |
About −15°C, bacterial growth detected; pure cultures, e.g., grow at less than −12°C |
Algae and protists in snow and ice; fish and invertebrates in −2°C Arctic water; −18°C, Himalayan midge |
Brine pockets in sea ice at about −30° |
Acid pH |
pH 0, acidophilic archaeans; e.g., Picrophilus sp. and Ferroplasma sp. |
pH 0, fungi, e.g., Cephalosporium; pH 0.5, acidophilic algae, e.g., Cyanidium caldarium, Dunaliella acidophila |
Acid mine drainage; geothermal sulfurous sites (e.g., Yellowstone) |
Alkaline pH |
pH 13, Plectonema; pH 10.5, Natrobacterium; pH 9-11, Methanosarcinales at Lost City hydrothermal vent environment |
pH 10, many species of protists and rotifers (e.g., Lake Nakuru, Africa); diatoms (e.g., Mono Lake) |
Soda lakes; peridotite-hosted hydrothermal systems (e.g., Lost City) |
High hydrostatic pressure |
High diversity of bacteria and archaeans in deep ocean trenches, including obligate pressure-requiring organisms (piezophiles) |
High diversity of invertebrates and fishes in ocean trenches |
11,100 m (Challenger Deep, Marianas Trench) |
Low water activity (desiccation) |
1. Grow in 35% NaCl, archaeans and bacteria, e.g., Halobacteria |
Molds and yeasts, e.g., Zygosaccharomyces rouxi (grow in high sugar or dried fruit, about 15-20% water) |
Deep-sea brines, soda lakes, evaporate ponds, dry soil and rocks, foods with high solute content (jams, honey, dried fruit) |
|
2. Survive dessication, e.g., Deinococcus sp. and Mycobacteria sp. |
Brine shrimp (Artemia sp.), cysts, tardigrades can survive dessication |
|
Damaging radiation (survival, not growth) |
10,000-11,000 grays (gamma radiation), Deinococcus radiodurans |
German cockroach (Blatella germanica) can survive exposure to radiation above 1,000 grays |
No natural source of radiation at level tolerated by D. radiodurans |
Toxic heavy metals |
Cd 2-5 mM, bacteria and archaeans; Ni 2.5 mM, Co 20 mM, Zn 12 mM, Cd 2.5mM, Ralstonia eutrophus |
Algae, e.g., Euglena and Chlorella can grow in Cd, Zn, and Co at mM concentrations |
Submarine hydrothermal vent fluids and sulfides; some high-metal-containing lakes |
SOURCES: Adapted from Cox, M.M., and Battista, J.R., 2005, Deinococcus radiodurans—The consummate survivor, Nat. Rev. Microbiol. 3:882-892; Deming, J.W., 2002, Psychrophiles and polar regions, Curr. Opin. Microbiol. 5:301-309; Holland, M., and Baross, J.A., 2003, Limits of life in hydrothermal systems, pp. 235-250 in Energy and Mass Transfer in Marine Hydrothermal Systems (P.E. Halbach, V. Tunnicliffe, and J. Hein, eds.), Proceedings of the 89th Dahlem Conference, Springer-Verlag, Berlin; López-García, P., 2005, Extremophiles, in Lectures in Astrobiology, Volume 1 (M. Garguad, B. Barbier, H. Martin, and J. Reisse, eds.), Springer-Verlag, Berlin; Potts, M., 1994, Desiccation tolerance of prokaryotes, Microbiol. Rev. 58:755-805; Rothschild, L.J., and Mancinelli, R.L., 2001, Life in extreme environments, Nature 409:1092-1101; Silver, S., and Phung, L.T., 1996, Bacterial heavy metal resistance: New surprises, Annu. Rev. Microbiol. 50:753-789; Ventoza, A., Nieto, J.J., and Oren, A., 1998, Biology of moderately halophilic aerobic bacteria, Microbiol. Mol. Biol. Rev. 62:504-544; Wiegel, J., and Kevbrin, V.V., 2003, Alkalithermophiles, Biochem. Soc. Trans. 32:193-198. |
a wide range of salt concentrations and maintain internal salt concentrations typical of nonhalophilic organisms (generally about 0.85 percent NaCl).22
Some combinations of extreme conditions apparently prevent cells from growing. For example, no organisms have been characterized that can grow in high salt concentrations at the upper and lower limits of temperature and pH. It is not known whether such combinations pose an insurmountable barrier or there has been insufficient sampling or a lack of assessable habitats with those combinations. For example, a marine environment with high temperature (up to 90°C) and high pH (up to 11) that was only recently discovered is teeming with microorganisms.23 Some other combinations known to exist in natural environments—such as high pressure, high salt, and low temperature—have rarely been studied.24 There are also combinations of extreme conditions that are known to have a synchronous effect on the growth or survival of cells that are not adapted to them. That is the case for hydrostatic pressure and temperature and for salt and temperature.25 Low temperature and high hydrostatic pressure affect cell processes in the same way; the result is that the minimal growth temperature of nonpiezophilic microorganisms increases with increasing pressure. Similarly, high salt concentrations will increase the minimal or decrease the maximal growth temperatures of nonhalophilic microorganisms.
Organisms have evolved a wide array of physiological and metabolic strategies for growing in nutrient-poor environments. In the open ocean—particularly in the deep-water column, in deep sediments, and in deep crustal environments—carbon and energy sources are extremely scarce (oligotrophic), but active microorganisms are present (although their growth rates may be extremely low). Recently, Pelagibacter ubique, a representative of one of the most cosmopolitan microorganisms in oligotrophic oceans, was found to grow only in the in situ micromolar concentrations of organic carbon. P. ubique has one of the smallest genomes, but it includes all the genes essential for existing as a free-living organism (growing without help from other organisms).26 P. ubique and related marine oligotrophs may help to set the lower limits of concentration of organic compounds that can support the growth of heterotrophs. P. ubique and related marine oligotrophs could serve as models for designing strategies to detect similar organisms in Europa’s ocean, the subsurface of Mars, or Lake Vostok (under the surface of the central Antarctic ice sheet) if it is determined that these ice-covered bodies of water contain low concentrations of dissolved organic compounds.
3.3
WATER, DESICCATION, AND LIFE IN NONAQUEOUS SOLVENTS
Water and temperature are the only single variables known to prevent growth and survival of organisms. Temperature and pressure together determine the boundary conditions for liquid water. The other physical and chemical factors that are thought of as presenting extreme conditions—such as pH, pressure, damaging radiation, and toxic metals—are life-prohibiting factors for most organisms but not all. Over the course of evolution, life has adapted to the entire terrestrial range of those variables (see Table 3.1). However, there are some combinations of physical and chemical conditions in which no organisms have been known to grow. They include environments that have both high salt (over 30 percent NaCl by weight) and low temperature (below 0°C), such as in sea-ice inclusions, and high salt (over 30 percent NaCl) and high temperature (over 90°C), known to exist in brine pools beneath the Red Sea and the Mediterranean Sea. Is life capable of growing in those combinations of stressors, or have we not looked hard enough in the appropriate environments?
Life as we know it is a series of aqueous chemical reactions. There is a point where intracellular water activitya decreases to the point where cells die. Desiccation causes DNA to break, lipids to undergo permanent phase changes, and proteins to crystallize, denature, and undergo condensation reactions.27,28 Saturated brine pools (35 percent salt; water activity, 0.75) are naturally occurring low-water-activity environments that are inhabited by bacteria, archaeans, eukaryotic algae, and brine shrimp. Organisms that grow or survive in dry environments or in solutions with low water activity, such as brines and syrups, match their internal water activity with that of their environment by accumulating compatible solutes, which can be ions, such as K+, or low-molecular-weight soluble organic compounds that do not interfere with the normal physiological functions of the cell.29 The obli-
gate halophilic archaeans (which cannot survive without high salt conditions) accumulate K+ and Cl+ ions in the cytoplasm to a concentration similar to that in the environment. That strategy requires evolutionary adaptations of intracellular macromolecules and of metabolic and biosynthetic processes to operate despite high salt concentrations. Most other microorganisms and eukaryotes deal with desiccation by accumulating compatible organic compounds that include organophosphorus compounds, β-amino acids, and glycerol. Some organisms survive desiccation by forming spores or cysts; others, such as the bacterium Deinococcus radiodurans, have mechanisms to repair damage to their DNA caused by desiccation. Both bacteria and eukaryotes can grow in Antarctic rocks that have liquid water for only short thaw periods.
An important issue regarding water is the degree to which it is required by carbon-based life or whether an organic substance could replace it as the primary solvent. Another issue is the ability of organisms to survive in environmental conditions that are outside their known limits for growth. These conditions usually involve decreasing the water content of the cell, as in bacterial and fungal spores and some animals, such as tardigrades (water bears). Those issues are important in assessing whether carbon-based life could exist in liquid methane or ethane pools on Titan, survive the harsh physical conditions that would be encountered during transport from one planet to another, or survive long periods in a completely desiccated state and retain the ability to grow if water were eventually introduced.
Is it possible for carbon-based life to exist in solvents other than liquid water? Many organic solvents—including alcohols, phenols, and toluene—are extremely toxic to microorganisms. The degree of antimicrobial action of a solvent depends on its hydrophobicity. The more hydrophobic the solvent, the more readily it can accumulate in cellular membranes. The toxicity of a solvent to cells is due to its ability to permealize the cell membrane, which results in the leakage of macromolecules, including RNA and proteins.30 Organic solvents kill most microorganisms, but some bacteria can tolerate solvents at relatively high concentrations. Two mechanisms of solvent tolerance have been identified: membranes’ limiting the diffusion of solvents into the cell and specialized efflux mechanisms that remove solvents that have diffused into the cell.
Can the carbon-based biochemistry of life as we know it function in organic solvents? Certainly, many enzymes function in organic solvents, and many organic reactions fundamental to biochemistry can occur in nonaqueous solvents.31,32 However, even enzymes that are active in organic solvents need some bound water to maintain their active structure. Moreover, water is important in other vital biochemical reactions during metabolism and biosynthesis, and water is a product of metabolic reactions. Scientists infer that carbon-based life is not likely to be able to adapt to a pure-solvent milieu unless it has some mechanism to form water from such solvents as alcohols or to produce all the necessary water de novo from biochemical reactions. Are those mechanisms possible, and is there evidence that bacteria could grow in some organic solvent, with or without trace levels of water?
Until recently, it was generally believed that external water passively diffuses through cell membranes. That is not necessarily true, and specific channels in bacterial membranes have been identified that function as pores for the transport of water. Named aquaporins, they can facilitate the rapid transport of water during osmotic stress.33 The presence of specific water-transport pores makes it imaginable that some organisms have organic-solvent-resistant membranes with specialized aquaporins that extract and selectively transport water at low concentrations from the solvent-water mixture. Moreover, it has been demonstrated with oxygen isotopes that up to 70 percent of the intracellular water in actively growing Escherichia coli is generated by metabolic processes, and not derived from the environment.34 That finding needs to be confirmed, but it leads to the question of whether it is possible for organisms to grow in intracellular water generated exclusively de novo or in water and solvent mixtures in which the concentration of water is 10 percent or less. A cell would have to overcome two obvious problems: If it is heterotrophic and requires transport of organic nutrients from an organic-solvent milieu, a cell will require a membrane that can differentially transport organic nutrients and prevent solvent toxicity. If the organism is a methanogen or some other chemolithoautotroph that requires carbon and energy sources that are gases, only a mechanism for passive diffusion through a solvent-resistant membrane will be necessary. Do such organisms exist, or can carbon-based organisms be engineered to grow under those conditions? Addressing the question would be worthwhile in the context of life in organic ponds on Titan or life in a gaseous milieu of CO2, H2, N2, and so on. (See Chapter 6 for a discussion of biochemical processes that are possible and in some cases favored in organic solvents as compared with water.) However, there is no information about the possible alternative membrane structures that would be stable and function optimally in organic solvents.
3.4
TEMPERATURE
Temperature is a fundamental thermodynamic characteristic that affects all biochemical reactions, in particular setting limits on life because temperature and pressure determine whether water is in the liquid phase. Given the presence of liquid water, what is the allowable range of temperatures for life? Microorganisms have been cultured and growth observed at temperatures as high as 121°C35 and as low as −15°C (Figure 3.1).36 There is even evidence of intact microorganisms with DNA and RNA in hydrothermal vent sulfides at temperatures exceeding 200°C.37 Eukaryotic organisms, however, are not known to live above 60°C. Despite the wide gap in maximal growth temperatures between prokaryotic and eukaryotic cells, eukaryotes share all other extremes of life with bacteria and archaeans, including growth in environments of high acidity, high salt, high pressure, and high concentrations of toxic metals (see Table 3.1).
Microorganisms that grow best at temperatures above 80°C are called hyperthermophiles. The maximal temperature at which growth of cultured hyperthermophiles occurs can vary from 80°C to 121°C, and the minimal growth temperature can vary from about 40°C to about 80°C, depending on the organism.38,39 Hyperthermophiles include bacteria and archaeans, aerobes and anaerobes, and heterotrophs and autotrophs. Some acidophiles (which grow at low pH), alkalophiles (which grow at high pH), and radiation-resistant organisms are also hyperthermophiles. Hyperthermophiles have protein and lipid structures that are adapted to high temperature. No generalizations can be made about why enzymes and other proteins are thermally stable, but there are some recurrent characteristics.40 Protein structures are stabilized at high temperature through amino acid substitutions and, most important, the increased use of disulfide bonds for structural stabilization.41 Heat-stable, ether-linked lipids are universal in hyperthermophilic archaeans and in some hyperthermophilic bacteria. Fundamental changes in protein and lipid structure compensate for the increased mobility and fluidity at high temperatures. All hyperthermophiles studied have a reverse gyrase that positively supercoiles DNA. (The DNA of all other organisms is negatively supercoiled.) Supercoiling with cationic proteins increases the thermal stability of DNA.42
Is 121°C the highest temperature for growth of life? The chemical properties of water and biological macromolecules are affected by high temperature. Theoretically, it should be possible to engineer biological macromolecules to maintain their three-dimensional structure with increasing temperature by compensating for temperature effects with higher pressure or with increasing salt concentrations. That appears to be the case with hyperthermophilic proteins.43,44 Important cofactors, such as adenosine triphosphate (ATP) and nicotine-adenine dinucleotide (NAD), are thermally labile (subject to chemical modifications to enable coping). Hyperthermophiles stabilize ATP and NAD by increasing their turnover rates (rates of synthesis), by employing extrinsic factors, such as high ion concentrations, or by using intrinsically more stable replacements.45 Neither protein, nor DNA, nor cofactor lability precludes life at higher temperatures, and the upper temperature for life is still to be determined.
Freezing temperatures can kill cells if ice crystals are formed internally. Cells survive freezing if frozen quickly; viable cells can be preserved for long periods if frozen quickly in liquid nitrogen (−196°C). Slow freezing or slow thawing of cells favors ice-crystal formation that can damage macromolecules and structural polymers and lead to death. Cellular adaptations for preventing ice-crystal formation during slow freezing include increasing intracellular solute concentrations (similar to low-water-activity adaptation), production of exopolysaccharides, and modification of lipids and proteins to increase the fluidity of membranes and mobility of the enzymes.
Is there a lower temperature limit for life? Microbial activity has been measured at −20°C in ice, and photosynthesis has been observed in Antarctic cryptoendolithic (living hidden in the rock) lichens at −20°C.46 Water can remain liquid at temperatures lower than 30°C in the presence of salts or other solutes and at even lower temperatures in combination with soluble organic solvents. Enzyme activity has been measured at −100°C in a mixture of methanol, ethylene glycol, and water.47 There is also evidence of electron transfer and enzyme activity at −80°C in a marine bacterium.48 The process of vitrification (liquid water moving directly into the glassy state without ice-crystal formation), facilitated by the presence of salts and exopolymers (insoluble high-molecular-weight organic compounds, usually carbohydrates) in the starting solution, may be essential for such activity. It is possible that there is no low-temperature limit for enzyme activity or even cell growth if a suitable solvent or solvent mixture is available.49
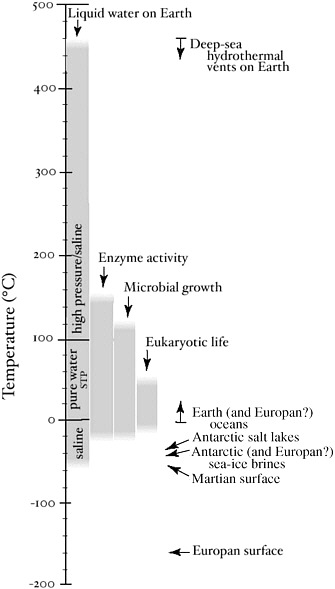
FIGURE 3.1 Liquid water and life on planets. Presence of liquid water and observed functioning on Earth of enzymes and microorganisms (Bacteria and Archaea). Note that more complex organisms (Eukarya) occupy a more restrictive thermal range. Average surface temperatures on Mars (−55°C) and Europa (−160°C) are also shown. SOURCE: Deming, J.W., and Eicken, H. Life in ice. In Planets and Life: The Emerging Science of Astrobiology (W. Sullivan III and J. Baross, eds.). Cambridge University Press, in press.
3.5
SURVIVAL STRATEGIES AND INTERPLANETARY TRANSFER
The ability of microorganisms to survive extreme environmental conditions—such as high and low temperature, desiccation, radiation, and extremes in pressures and pH—increases the probability that they could survive transport to other planets and moons and thereby effect panspermia, the transfer of life from one world to another. Interplanetary transfer of life would be likely only within a solar system, and transfer to a planet orbiting another star would be extremely unlikely. Panspermia is an important issue in the context of this report because the presence of life in any extraterrestrial setting would require that it have an origin—de novo or by panspermia. Moreover, if panspermia is how life began on Earth, it is likely to be how life could arise elsewhere, and life forms found elsewhere would then resemble Earth life at the fundamental, biochemical level. Two groups of microorganisms have received most of the attention as possible successful space travelers: spore-forming bacteria and radiation-resistant microorganisms. Spore formation by bacteria and fungi is usually in response to stress, such as in limiting of nutrients, dessication, and heat shock. Spores are capable of long-term survival and have been recovered from environmental samples that are more than 1 million years old.50 There is even a report of viable spores from the gut contents of a bee entombed in amber estimated to be more than 25 million years old.51 Spores are also known to be resistant to heat and radiation; and, because of their small size and stable dormancy, they can travel through the atmosphere to distant locations or slowly settle on the floors of deep ocean trenches. Some spores can survive more than an hour of exposure to dry heat at 150°C, although survival is greatly reduced in moist heat.52 Spores from thermophilic bacteria are more resistant to heat than are mesophilic spores.53 Similarly, spores are much more resistant to ultraviolet radiation than are vegetative cells (those capable of growing).54
If microorganisms are to be transported from one planet to another, they must have the ability to resist the lethal effects of radiation. High-energy electromagnetic radiation (ultraviolet, x-ray, and gamma radiation) and high-energy alpha and beta particles damage DNA and have cytotoxic and mutagenic effects on cells. Ultraviolet radiation is the most abundant form of damaging radiation and probably the most common natural mutagen. Ultraviolet light can also kill cells as a result of dimerization of thymidine residues in the DNA, which prevents replication. Ionizing radiation kills cells by causing multiple breaks in the double-stranded DNA. Most organisms have mechanisms for protection from damaging radiation, such as radiation-absorbing pigments and DNA-repair mechanisms.
The most radiation-resistant microorganisms include bacteria and archaeans. Deinococcus radiodurans is one of the most studied of those organisms and can survive radiation levels up to 10 kGy.b That level of radiation is much higher than is found naturally anywhere on Earth. Radiation resistance in D. radiodurans is the result of extremely efficient DNA-repair mechanisms that can reassemble, with high fidelity, DNA that has been sheared into multiple double-stranded pieces.55 It is believed that this repair mechanism evolved in response to DNA damage due to desiccation rather than radiation. Recently, a hyperthermophilic archaean, from a submarine hydrothermal vent environment, was found to be resistant to radiation at a level of 8 kGy.56 The German cockroach (Blattella germanica) is the most radiation-resistant metazoan, although the tardigrade (Milnesium tardigradum) in its desiccation-resistant “tun” state can survive high-level exposures to x rays.
Besides being desiccated and irradiated, microorganisms traveling in space will be exposed to space vacuum that can reach 10−14 pascal (a unit of pressure—100 Pa = 1 mbar).57 The result is extreme dehydration, and naked spores can survive for only days if exposed to space vacuum. Survival of spores is increased if they are associated with various chemicals such as sugars, or are embedded in salt crystals. Nicholson et al. (2000) discuss the various stresses that a microbial cell or spore would have to endure to survive interplanetary travel.58 They include the process that transports them out of Earth’s atmosphere, such as volcanic eruptions and bolide impacts, long periods of transit in the cold of space, and atmospheric entry into a new planetary home. Spores have been shown to survive the shock conditions of a meteorite impact and the ultraviolet radiation and low temperature of space.59 It is clear that panspermia is possible and even probable if bacterial spores become embedded in rocks that are ejected from one planet and eventually enter the atmosphere of another. Bacterial
spores have received most of the attention with respect to panspermia, but they may not be the only candidates for long-term survival in space. There is evidence that microorganisms that are attached to surfaces, such as minerals, have enhanced survival of a variety of stress conditions.60 In many cases, the attached organisms form biofilms in which the cells are encapsulated in exopolysaccharides and often reach high densities.61 Stress resistance has been attributed to two features of the biofilm communities: their ability to limit rates of diffusion, and the presence of multiple physiological states, including “persister” cells with recalcitrant physiologies.62 More research is needed to assess the full range of microorganisms that can survive interplanetary travel, particularly those known to form biofilms on minerals.
3.6
THE PLASTICITY OF HUMAN-LIKE BIOCHEMISTRY
As the exploration of the biosphere has continued, environments on Earth have been discovered that are quite different from and alien to human-like life. Nevertheless, where an environment has been found to contain a chemical disequilibrium, if liquid water is also present and the temperature does not exceed the upper limit for covalent bonding of core biomolecules, life is present. Active life has been found in deep-ocean thermal vents at temperatures in excess of 112°C. Life has been found in Antarctica where liquid water exists only transiently. It has been found 5 km below Earth’s surface in mine shafts, and in the effluents of mining operations at Rio Tinto, Spain, that are as acidic as dilute sulfuric acid. Several of those environments are summarized in Table 3.1.
The life that is present in those environments is, in many of its molecular aspects, identical with human life. It exploits the same DNA for genetics, the same amino acids in its proteins, and the same core metabolism. Furthermore, analysis of its chemical structure leaves no doubt that these exotic terran life forms are related to humans by common ancestry.
Those observations compel the conclusion that the standard macromolecular, genetic, catalytic, and metabolic structures are versatile enough to support life in exotic environments. How life adapted to such environments and what its primitive environment was remain topics of research. The details of how human-like forms of life have adapted to exotic environments give some clues to those questions and suggest environments where human-like life could not survive.
3.7
LIMITS OF ANTHROPOCENTRIC BIOCHEMISTRY
As plastic as standard terran biochemistry is, it is easy to find on Earth environments with features whose exotic or extreme nature cannot be directly accommodated by human-like biomolecules. For example, the nucleobases of DNA and RNA are, as their name implies, chemical bases. They can absorb a proton from their environment to become positively charged. In adenine and cytosine, for example, the nucleobases are more than 50 percent protonated below a pH of 4, the acidity of dilute vinegar. That would argue against an origin of life in an acidic environment.
Because the protonated forms of adenine and cytosine have different arrangements of hydrogen atoms, they form different patterns of hydrogen bonding. Thus, for example, adenine is no longer complementary to thymine, and cytosine is no longer complementary to guanine. That, in turn, means that the Watson-Crick nucleobase pairing essential for DNA duplex formation and genetics is no longer possible.
The motion of protons in water is extremely rapid, and no catalyst can manage this problem at low pH. Therefore, the known life that is found in acidic environments on Earth manages the low pH of its environment by pumping protons out of the cell. Although the pH of Rio Tinto outside the cells that live there is about 2, the pH inside the cells is well above 6, as it is in human cells.
The same considerations limit the intrinsic adaptability of standard terran biochemistry at high pH.63 Guanine and thymidine and the RNA analogue uracil are weak acids. They lose a proton to water at a pH above 10. The deprotonated forms of those nucleobases have different arrangements of hydrogen atoms and form different patterns of hydrogen bonding. That means that they are no longer complementary to their standard Watson-Crick partners, and that the nucleobase pairing essential for DNA duplex formation and genetics is thus no longer possible.
Any forms of life that are found at high pH must either pump protons to maintain an intracellular pH close to neutrality or adopt a genetic structure that is different from the chemical structures found in standard DNA. The first is the strategy for organisms that live in such alkaline environments as Lake Mono.
High pH has other limits as well. The hydroxide anion, arising from water at a high pH, is a powerful nucleophile and destroys many molecules that are part of core metabolism. Thioesters have short half-lives in neutral water; their half-lives at a pH of 12 are measured in seconds. They play central roles in the manipulation of organic acids, including the formation of new organic acids by the formation of new carbon-carbon bonds and the degradation of organic acids through the breakage of carbon-carbon bonds.
The second set of organic molecules that become unacceptably unstable at high pH are the mixed anhydrides of organic acids and phosphoric acid. They are central in the management of high-energy phosphate esters in living systems, including the generation of ATP from glucose.
At still higher pH, standard oxygen esters, such as those found in lipids (see Figure 2.12), become unacceptably unstable. Standard oxygen esters are key components of many hydrophobic structures, including membrane structures in normal bacteria. That is why human skin, for example, suffers a reaction when it comes into contact with lye. It would be difficult to imagine life containing fatty acid esters surviving, for example, in water-ammonia eutectics on Titan. An alternative hydrophobic system would be required.
It is clear that biological systems can manage the chemical reactivity of unstable species. For example, oxaloacetate—a metabolic intermediate in terran metabolism that is a precursor of citric acid, malic acid, and the amino acid aspartic acid—decarboxylates readily, with a half-life measured in minutes at room temperature at neutral pH. The half-life for the decarboxylation of oxaloacetate drops to seconds at high temperatures in pure water. It is not clear how microorganisms that live at high temperatures manage the instability of oxaloacetate, which is a key intermediate in standard biochemistry for the formation of amino acids, such as aspartate, and asparagine.
3.8
EARLY ENVIRONMENTS OF LIFE ON EARTH
That RNA and DNA could not work well if bathed in water having a pH much higher than 9 or much lower than 5 suggests that these are the bounds for the pH of the environment where RNA and DNA first functioned as genetic molecules. If we assume that the most primitive forms of life on Earth could not have been sophisticated enough to pump protons across membranes that would not leak protons and if we assume that RNA and DNA worked in the first living systems, we are compelled to conclude that the first living systems lived at a pH near 7. An alternative possibility is that RNA and DNA were first used by terran organisms after they evolved sufficiently to control their internal pH. How replication would have occurred until that happened remains uncertain.
Other constraints on the temperature at which earlier forms of life lived come from experiments. For example, Gaucher et al.64 compared the sequences of elongation factors from a variety of bacteria to infer the sequences of ancestral elongation factors that may have been present in now-extinct bacteria lying deep in the eubacterial tree. With recombinant-DNA technology, several of those candidate ancestral elongation factors were resurrected for study in the laboratory. They were found to function best at a temperature of about 67°C, typical for modern thermophilic bacteria.
Despite their antiquity (perhaps 2.5 billion years old), the resurrected ancestral elongation factors are inferred for bacteria that lived long after the origin of life. Nevertheless, the notion that early life lived at high temperatures, in water, and at nearly neutral pH is consistent with available data. For example, a recent reconstruction of the phylogenetic tree of life based on 31 common gene families supports the notion that the last common ancestor lived at high temperatures.65
3.9
OPPORTUNITIES FOR RESEARCH
The exploration of the terran biosphere has been a continuous source of insights and surprises, even to the present date. The development of high-throughput DNA sequencing techniques and sequencing surveys of habitat microorganisms makes it possible to continue to expand our understanding of the inventory of metabolic diversity on the planet. Molecular methods are not likely to find any life that is not related by common ancestry to the life
that we already know, and so it remains important to continue to push our search for the limits of life on Earth. For example, if polyelectrolytes are universal features of genetic biopolymers, the search for them might uncover life forms that are not identified with standard polymerase chain reaction primers directed at ribosomal RNA genes.66 Laboratory research and field studies are needed so that we would recognize, on Earth, life that is not related to us by common ancestry.67 Concepts and instruments that are designed to do that would be applicable to flight missions as well.
Carbon-based life is extremely versatile and has adapted to grow in most of the physicochemical environments on Earth. Only high temperatures and low water activities limit the growth of organisms. Even in those limiting conditions, some organisms have adapted strategies for long-term survival.
There is still much to be understood about the limits of carbon-based life. Some extreme terran environments have not been adequately explored for microbes or for evidence of microbial interactions. They include the hot subseafloor crust, hot brine sediments and crustal aquifers, and rock-hosted microbial communities, particularly those affected by hydrothermal activity or subjected to changing environmental extremes, such as hydrating and dehydrating conditions. Even in relatively well-studied environments, including extreme environments, very little is known about the microbial inhabitants other than their identities based on molecular signatures. There is also a need to understand how different physiological characteristics of organisms, such as biofilm formation and production of exopolysaccharides, affect growth and survival in extreme environments. It is also probable that the limits of extant terran organisms might not be indicative of limits possible for organisms with terran biochemistry. Life occupies most known habitats and in some cases can tolerate extreme conditions, such as irradiation and hydrostatic pressure, that exceed those found in natural environments. Important unanswered questions include these: What is the maximal growth temperature for life? Are there extrinsic or intrinsic factors—such as high hydrostatic pressure, high concentrations of salt, or high concentrations of intracellular inorganic and organic compounds and macromolecules—that facilitate growth above 120°C? Can organisms adapt to grow with much lower amounts of water or higher concentrations of organic solvents than are now accepted as limits? The ability of cells to grow under such conditions would change our perception of the lower and upper temperature limits for life. Can organisms grow in environments that are deficient in one or more essential nutrients, such as phosphate, by substituting arsenic, for example?
3.10
REFERENCES
1 Cox, M.M., and Battista, J.R. 2005. Deinococcus radiodurans—The consummate survivor. Nature Rev. Microbiol. 3:882-892.
2 Sharma, A., Scott, J.H., Cody, G.D., Fogel, M.L., Hazen, R.M., Hemley, R.J., and Huntress, W.T. 2002. Microbial activity at gigapascal pressures. Science 295:1514-1516.
3 Wackett, L.P., Dodge, A.G., and Ellis, L.B.M. 2004. Microbial genomics and the periodic table. Appl. Environ. Microbiol. 70:647-655.
4 Beatty, J.T., Overmann, J., Lince, M.T., Manske, A.K., Lang, A.S., Blankenship, R.E., Van Dover, C.L., Martinson, T.A., and Plumley, F.G. 2005. An obligately photosynthetic bacterial anaerobe from a deep-sea hydrothermal vent. Proc. Natl. Acad. Sci. U.S.A. 102(26):9306-9310.
5 Huber, H., Hohn, M.J., Rachel, R., Fuchs, T., Wimmer, V.C., and Stetter, K.O. 2002. A new phylum of Archaea represented by a nanosized hyperthermophilic symbiont. Nature 417:63-67.
6 Smith, H.O., Hutchison III, C.A., Pfannkoch, C., and Venter, J.C. 2003. Generating a synthetic genome by whole genome assembly: ØX174 bacteriophage from synthetic oligonucleotides. Proc. Natl. Acad. Sci. U.S.A. 100:15440-15445.
7 Feinberg, G., and Shapiro, R. 1980. Life Beyond Earth: The Intelligent Earthling’s Guide to Life in the Universe. New York: William Morrow.
8 Schulze-Makuch, D., and Irwin, L.N. 2004. Life in the Universe: Expectations and Constraints. Berlin: Springer-Verlag.
9 Hallsworth, J.E., Yakimov, M.M., Golyshin, P.N., Gillion, J.L.M., D’Auria, G., de Lima Alves, F., La Cono, V., Genovese, M., McKew, B.A., Hayes, S.L., Harris, G., Giuliano, L., Timmis, K.N., and McGenity, T.J. 2007. Limits of life in MgCl2-containing environments: Chaotropicity defines the window. Environ. Microbiol. 9(3):801-813.
10 Navarro-González, R., Rainey, F.A., Molina, P., Bagaley, D.R., Hollen, B.J., de la Rosa, J., Small, A.M., Quinn, R.C., Grunthaner, F.J., Cáceres, L., Gomez-Silva, B., and McKay, C.P. 2003. Mars-like soils in the Atacama Desert, Chile, and the dry limit of microbial life. Science 302:1018-1021.
11 Kelley, D.S., Baross, J.A., and Delaney, J.R. 2002. Volcanoes, fluids, and life at mid-ocean ridge spreading centers. Annu. Rev. Earth Planet. Sci. 30:385-491.
12 Baross, J.A., and Deming, J.W. 1998. Growth at high temperatures: Isolation and taxonomy, physiology, and ecology. Pp. 169-217 in The Microbiology of Deep-Sea Hydrothermal Vents (D.M. Karl, ed.). CRC Press, Boca Raton, Fla.
13 López-García, P. 2005. Extremophiles. In Lectures in Astrobiology, Volume 1 (M. Garguad, B. Barbier, H. Martin, and J. Reisse, eds.). Springer-Verlag, Berlin.
14 Rothschild, L.J., and Mancinelli, R.L. 2001. Life in extreme environments. Nature 409:1092-1101.
15 Holland, M., and Baross, J.A. 2003. Limits of life in hydrothermal systems. Pp. 235-250 in Energy and Mass Transfer in Marine Hydrothermal Systems (P.E. Halbach, V. Tunnicliffe, and J. Hein, eds.). Proceedings of the 89th Dahlem Conference. Springer-Verlag, Berlin.
16 Bartlett, D.H. 2002. Pressure effects on in vivo microbial processes. Biochemica et Biophysica Acta 1595:367-381.
17 Horikoshi, K. 1999. Alkaliphiles: Some applications of their products for biotechnology. Microbiol. Mol. Biol. Rev. 63:735-750.
18 Silver, S., and Phung, L.T. 1996. Bacterial heavy metal resistance: New surprises. Annu. Rev. Microbiol. 50:753-789.
19 Wiegel, J., and Kevbrin, V.V. 2003. Alkalithermophiles. Biochem. Soc. Trans. 32:193-198.
20 Ventoza, A., Nieto, J.J., and Oren, A. 1998. Biology of moderately halophilic aerobic bacteria. Microbiol. Mol. Biol. Rev. 62:504-544.
21 Ciaramella, M., Napoli, A., and Rossi, M. 2004. Another extreme genome: How to live at pH 0. TRENDS Microbiol. 13:49-51.
22 Ventoza, A., Nieto, J.J., and Oren, A. 1998. Biology of moderately halophilic aerobic bacteria. Microbiol. Mol. Biol. Rev. 62:504-544.
23 Kelley, D.S., Karson, J.A., Früh-Green, G.L., Yoerger, D.R., Shank, T.M., Butterfield, D.A., Hayes, J.M., Schrenk, M.O., Olson, E.J., Prokurowski, G., Jakuba, M., Bradley, A., Larson, B., Ludwig, K., Glickson, D., Buckman, K., Bradley, A.S., Brazelton, W.J., Roe, K., Elend, M.J., Delacour, A., Bernasconi, S.M., Lilley, M.D., Baross, J.A., Summons, R.E., and Sylva, S.P. 2005. A serpentinite-hosted ecosystem: The lost city hydrothermal field. Science 307:1428-1434.
24 Kaye, J.Z., and Baross, J.A. 2004. Synchronous effects of temperature, pressure and salinity on growth, phospholipid profiles, and protein patterns of four Halomonas species isolated from deep-sea hydrothermal-vent and sea surface environments. Appl. Environ. Microbiol. 70:6220-6229.
25 Kaye, J.Z., and Baross, J.A. 2004. Synchronous effects of temperature, pressure and salinity on growth, phospholipid profiles, and protein patterns of four Halomonas species isolated from deep-sea hydrothermal-vent and sea surface environments. Appl. Environ. Microbiol. 70:6220-6229.
26 Giovannoni, S.J., Tripp, H.J., Givan, S., Podar, M., Vergin, K.L., Baptista, D., Bibbs, L., Eads, J., Richardson, T.H., Noordewier, M., Rappé, M.S., Short, J.M., Carrington, J.C., and Mathur, E.J. 2005. Genome streamlining in a cosmopolitan oceanic bacterium. Science 309:1242-1245.
27 Billi, D., and Potts, M. 2002. Life and death of dried prokaryotes. Res. Microbiol. 153:7-12.
28 Potts, M. 1994. Desiccation tolerance of prokaryotes. Microbiol. Rev. 58:755-805.
29 Müller, V., Spanheimer, R., and Santos, H. 2005. Stress response by solute accumulation in archaea. Curr. Opin. Microbiol. 8:729-736.
30 Isken, S., and de Bont, J.A.M. 1998. Bacteria tolerant to organic solvents. Extremophiles 2:229-238.
31 Benner, S.A., Ricardo, A., and Carrigan, M.A. 2004. Is there a common chemical model for life in the universe? Curr. Opin. Chem. Biol. 8:672-689.
32 Bragger, J.M., Dunn, R.V., and Daniel, R.M. 2000. Enzyme activity down to 100°C. Biochim. Biophys. Acta 1480:278-282.
33 Stroud, R.M., Miercke, L.J.W., O’Connell, J., Khademi, S., Lee, J.K., Remis, J., Harries, W., Robles, Y., and Akhavan, D. 2003. Glycerol facilitator GlpF and the associated aquaporin family of channels. Curr. Opin. Struct. Biol. 13:424-431.
34 Kreuzer-Martin, H.W., Ehleringer, J.R., and Hegg, E.L. 2005. Oxygen isotopes indicate most intracellular water in log-phase Escherichia coli is derived from metabolism. Proc. Natl. Acad. Sci. U.S.A. 102:17337-17341.
35 Kashefi, K., and Lovley, D.R. 2003. Extending the upper temperature limit for life. Science 301:934.
36 Junge, K., Eicken, H., and Deming, J.W., 2004, Bacterial activity at 2 to 20°C in Arctic wintertime sea ice, Appl. Environ. Microbiol. 70:550-557; Kashefi, K., and Lovley, D.R., 2003, Extending the upper temperature limit for life, Science 301:934.
37 Schrenk, M.O., Kelley, D.S., Delaney, J.R., and Baross, J.A., 2003. Incidence and diversity of microorganisms within the walls of an active deep-sea sulfide chimney. Appl. Environ. Microbiol. 69:3580-3592.
38 Holden, J.F., and Daniel, R.M. 2004. The upper temperature for life based on hyperthermophile culture experiments and field observations. Pp. 13-24 in The Subseafloor Biosphere at Mid-Ocean Ridges (W.S.D. Wilcock, E.F. DeLong, D.S. Kelley, J.A. Baross, and S.C. Cary, eds.). Geophysical Monograph 144. American Geophysical Union, Washington D.C.
39 Kashefi, K., and Lovley, D.R. 2003. Extending the upper temperature limit for life. Science 301:934, Table 1.
40 Charlier, D., and Droogmans, L. 2005. Microbial life at high temperatures, the challenges, the strategies. Cell. Mol. Life Sci. 62:2974-2984.
41 Beeby, M., O’Connor, B.D., Ryttersgaard, C., Boutz, D.R., Perry, L.J., and Yeates, T.O. 2005. The genomics of disulfide bonding and protein stabilization in thermophiles. PloS Biology 3:1549-1558.
42 Daniel, R.M., Holden, J.F., van Eckert, R., Truter, J., and Cowan, D.A. 2004. The stability of biomolecules and the implications for life at high temperatures. Pp. 25-39 in The Subseafloor Biosphere at Mid-Ocean Ridges (W.S.D. Wilcock, E.F. DeLong, D.S. Kelley, J.A. Baross, and S.C. Cary, eds.). Geophysical Monograph 144. American Geophysical Union, Washington D.C.
43 Sterner, R., and Liebl, W. 2001. Thermophilic adaption of proteins. Crit. Rev. Biochem. Mol. Biol. 36:39-106.
44 Summit, M., Scott, B., Nielson, K., Mathur, E., and Baross, J.A. 1998. Pressure enhances thermal stability of DNA polymerase from three thermophilic organisms. Extremophiles 2:339-345.
45 Daniel, R.M., Holden, J.F., van Eckert, R., Truter, J., and Cowan, D.A. 2004. The stability of biomolecules and the implications for life at high temperatures. Pp. 25-39 in The Subseafloor Biosphere at Mid-Ocean Ridges (W.S.D. Wilcock, E.F. DeLong, D.S. Kelley, J.A. Baross, and S.C. Cary, eds.). Geophysical Monograph 144. American Geophysical Union, Washington D.C.
46 Friedmann, E.I., and Sun, H.J. 2005. Communities adjust their temperature optima by shifting producer-to-consumer ratio, shown in lichens as models: 1. Hypothesis. Microb. Ecol. 49:523-527.
47 Bragger, J.M., Dunn, R.V., and Daniel, R.M. 2000. Enzyme activity down to −100°C.Biochim. Biophys. Acta 1480:278-282.
48 Junge, K, Eicken, H., Swanson, B.D., and Deming, J.W. 2006. Bacterial incorporation of leucine into protein down to −20°C with evidence for potential activity in subeutectic saline ice formations. Cryobiology 52(3):417-429.
49 Price, B., and Sowers, T. 2004. Temperature dependence of metabolism rates for microbial growth, maintenance, and survival. Proc. Natl. Acad. Sci. U.S.A. 101:4631-4636.
50 Dmitriev, W., Suzina, N.E., Barinova, E.S. Duda, V.I., and Boronin, A.M. 2004. An electron microscopic study of the ultrastructure of microbial cells in extreme biotopes in situ. Microbiol. 73:716-723.
51 Cano, R.J., and Borucki, M.K. 1995. Revival and identification of bacterial spores in 25- to 40-million-year-old Dominican amber. Science 268:1060-1064.
52 Nicholson, W.L., Munakata, N., Horneck, G., Melosh, H.J., and Setlow, P. 2000. Resistance of Bacillus endospores to extreme terrestrial and estraterrestrial environments. Microbiol. Mol. Biol. Rev. 64:548-572.
53 Ashton, D., and Bernard, D. 1992. Thermophilic anaerobic sporeformers. Pp. 309-316. Compendium of Methods for the Microbiological Examination of Foods, 3rd Edition (C. Vanderzant and D.F. Splittstoesser, eds.). American Public Health Association, Washington, D.C.
54 Nicholson, W.L., Munakata, N., Horneck, G., Melosh, H.J., and Setlow, P. 2000. Resistance of Bacillus endospores to extreme terrestrial and estraterrestrial environments. Microbiol. Mol. Biol. Rev. 64:548-572.
55 Cox, M.M., and Battista, J.R. 2005. Deinococcus radiodurans—The consummate survivor. Nature Rev. Microbiol. 3:882-892.
56 Jolivet, E., Corre, E., L’Haridon, S., Forterre, P., and Prieur, D. 2004. Thermococcus marinus sp. nov. and Thermococcus radiotolerans sp. nov., two hyperthermophilic archaea from deep-sea hydrothermal vents that resist ionizing radiation. Extremophiles 8:219-227.
57 Nicholson, W.L., Munakata, N., Horneck, G., Melosh, H.J., and Setlow, P. 2000. Resistance of Bacillus endospores to extreme terrestrial and estraterrestrial environments. Microbiol. Mol. Biol. Rev. 64:548-572.
58 Nicholson, W.L., Munakata, N., Horneck, G., Melosh, H.J., and Setlow, P. 2000. Resistance of Bacillus endospores to extreme terrestrial and estraterrestrial environments. Microbiol. Mol. Biol. Rev. 64:548-572.
59 Horneck, G., Mileikowsky, C., Melosh, H.J., Wilson, J.W., Cucinotta, F.A., and Gladman, B. 2002. Viable transfer of microorganisms in the solar system and beyond. Pp. 57-76 in Astrobiology: The Quest for the Conditions of Life (G. Horneck and C. Baumstark-Khan, eds.). Springer, Berlin.
60 Edwards, K.J., Bach, W., and McCollom, T.M., 2005, Geomicrobiology in oceanorgraphy: Microbe-mineral interactions at and below the seafloor, Trends in Microbiol. 13:449-456; Schrenk, M.O., Kelley, D.S., Delaney, J.R., and Baross, J.A., 2003, Incidence and diversity of microorganisms within the walls of an active deep-sea sulfide chimney, Appl. Environ. Microbiol. 69:3580-3592; Schrenk, M.O., Kelley, D.S., Bolton, S., and Baross, J.A., 2004, Low archaeal diversity linked to sub-seafloor geochemical processes at the Lost City Hydrothermal Field, Mid-Atlantic Ridge, Environ. Microbiol. 6:1086-1095.
61 Branda, S.S., Vik, A., Friedman, L., and Kolter, R. 2005. Biofilms: The matrix revisited. Trends in Microbiol. 13:20-26; Parsek, M.R., and Greenberg, E.P. 2005. Sociomicrobiology: The connections between quorum sensing and biofilms. Trends Microbiol. 13:27-33.
62 Wimpenny, J. 2000. Heterogeneity in biofilms. FEMS Microbiol. Rev. 24:661-671.
63 Saenger, W. 1988. Principles of Nucleic Acid Structure. Springer-Verlag, New York, pp. 114-115.
64 Gaucher, E.A., Thomson, J.M., Burgan, M.F., and Benner, S.A. 2003. Inferring the paleoenvironment during the origins of bacteria based on resurrected ancestral proteins. Nature 425:285-288.
65 Ciccerelli, F.D., Doerks, T., von Mering, C., Creevey, C.J., Snel, B., and Bork, P. 2006. Toward automatic reconstruction of a highly resolved tree of life. Science 311:1283-1287.
66 Benner, S.A., Hutter, D. 2002. Phosphates, DNA, and the search for nonterrean life: A second generation model for genetic molecules. Bioorg. Chem. 30:62-80.
67 Davies, P.C.W., and Lineweaver, C.H. 2005. Finding a second sample of life on Earth. Astrobiology 5:154-163.