9
Vaccines
WHERE WE WANT TO BE IN THE YEAR 2010
After years of research, several different malaria vaccines will be available and licensed for use in humans. For infants and children in highly endemic regions of the world, an inexpensive, safe, and stable vaccine that gives long-lasting protection from death and severe clinical illness will be integrated into existing immunization programs. For nonimmune visitors to malarious areas and for the control of epidemics, there will be a vaccine that gives complete short-term protection against malaria with few side effects. Finally, a vaccine that confers no individual protection, but prevents the development of the parasite in the mosquito, will be used in combination with the other vaccines and control measures to reduce, and in some areas even interrupt, malaria transmission.
WHERE WE ARE TODAY
Prospects for a Vaccine
Vaccination is an exceptionally attractive strategy for preventing and controlling malaria. Clinical and experimental data support the feasibility of developing effective malaria vaccines. For example, experimental vacci-
nation with irradiated sporozoites can protect humans against malaria, suggesting that immunization with appropriate sporozoite and liver-stage antigens can prevent infection in individuals bitten by malaria-infected mosquitoes. In addition, repeated natural infections with the malaria parasite induce immune responses that can prevent disease and death in infected individuals, and the administration of serum antibodies obtained from repeatedly infected adults can control malaria infections in children who have not yet acquired protective immunity. These data suggest that immunization with appropriate blood-stage antigens can drastically reduce the consequences of malaria infection. Finally, experimental evidence shows that immunization with sexual-stage antigens can generate an immune response that prevents parasite development in the vector, offering a strategy for interrupting malaria transmission.
Prospects for the development of malaria vaccines are enhanced by the availability of suitable methods for evaluating candidate antigens. These include protocols that allow human volunteers to be safely infected with malaria, and the identification of many areas in the world where more than 75 percent of individuals can be expected to become infected with malaria during a three-month period. In contrast to vaccines for diseases of low incidence, for which tens of thousands of immunized and nonimmunized controls must be studied over several years, malaria vaccines could be evaluated in selected areas in fewer than 200 volunteers in less than a year.
Developments in molecular and cellular biology, peptide chemistry, and immunology provide the technological base for engineering subunit vaccines composed of different parts of the malaria parasite, an approach that was not possible 10 years ago. During the last 5 years, more than 15 experimental malaria vaccines have undergone preliminary testing in human volunteers. Although none of these vaccines has proven suitable for clinical implementation, progress has been made in defining the parameters of a successful vaccine and the stage has been set for further advancement.
Despite the inherent attractiveness and promise of this approach, there remain a number of obstacles to vaccine development. With the exception of the erythrocytic (blood) stages of P. falciparum, human malaria parasites cannot be readily cultured in vitro, limiting the ability of researchers to study other stages of this parasite and all stages of the other three human malaria parasite species.
In vitro assays, potentially useful for screening candidate vaccines for effectiveness, do not consistently predict the level of protective immunity seen in vivo. The only laboratory animals that can be infected with human malaria parasites are certain species of nonhuman primates, which are not naturally susceptible to these organisms. This makes it difficult to com-
pare the results of many studies done in animals with what happens in human malaria infection.
The promises of modern vaccinology, while potentially revolutionary, have so far proved elusive. Few commercially available vaccines have been produced by this technology, for both scientific and economic reasons. Scientists have not yet been able to assemble defined synthetic peptides and recombinant proteins and combine them with new adjuvants and delivery systems into a practical human malaria vaccine. However, as discussed above and in the remainder of this chapter, there are good reasons to believe that this approach will ultimately succeed.
Approaches to Vaccine Development
The complex life cycle of the malaria parasite provides a number of potential targets for vaccination (Figure 9-1). Under investigation are vaccines that would be effective against the extracellular sporozoite, during the short period it spends in the bloodstream; the exoerythrocytic (or liver-stage) parasite, during the roughly seven days it develops within liver cells; the extracellular merozoite, released from liver cells or infected erythrcytes and
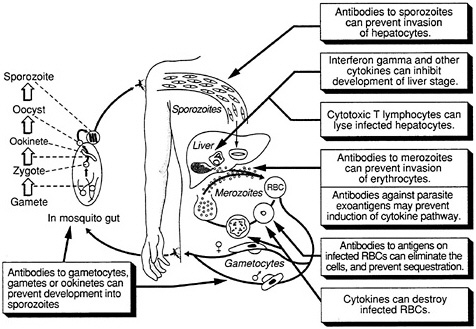
FIGURE 9-1 Host defense against malaria. (Adapted, with permission, from Rickman, L. S., and S. L. Hoffman. 1991. Malaria. Pg. 1039 in Medical Microbiology, 3rd Ed., S. Baron, ed. New York: Churchill Livingstone)
free in the circulation prior to invading other erythrocytes; the asexual parasite that develops within red blood cells; exogenous parasite material released from infected erythrocytes; and the sexual-stage parasite, which occurs both inside erythrocytes and in mosquitoes. The optimal vaccine would include antigens from the sporozoite, asexual, and sexual stages of the parasite, thus providing multiple levels of control, but vaccines effective against individual stages could also prove highly useful. In addition, a vaccine against the Anopheles mosquito itself, which reduced the insect's life span and prevented complete development of the parasite, could be valuable.
Regardless of the stage of parasite targeted for vaccine development, a similar strategy is envisioned. Based on knowledge of the mechanisms of protective immunity, specific parasite antigens (immunogens) are identified that induce a protective immune response, and synthetic or recombinant vaccines that accurately mimic the structure of that antigen are prepared.
In the subunit approach to vaccine development, this is done by combining the immunogen with carrier proteins, adjuvants, and live vectors or other delivery systems. This approach is being pursued throughout the world in laboratories studying infectious diseases. Clinical utility has yet to be demonstrated for the majority of these efforts, and barriers to obtaining satisfactory immunization by the subunit approach remain. Nevertheless, research on malaria subunit vaccines will continue to be at the cutting edge of this innovative and important approach to vaccine development.
Pre-Erythrocytic Vaccines
A pre-erythrocytic vaccine is designed to prevent malaria infection. If all sporozoites and liver stages of the parasite are destroyed before they can mature to cause blood-stage infection, all clinical manifestations of malaria will be prevented. Such a vaccine, even if it induced protection that lasted for only a few months, would be especially useful for tourists, diplomats, businessmen, military personnel, and other short-term visitors to malarious areas, since nonimmune individuals are highly susceptible to the rapid development of severe and fatal malaria. A pre-erythrocytic vaccine could be useful for long-term residents of malarious areas if it induced long-lasting protection, or if immunity could be boosted by natural exposure to malaria.
Mechanisms of Immunity
In the 1960s and 1970s a number of researchers showed that immunization with live sporozoites that were treated in such a way as to be noninfective
(attenuated) confers protection against subsequent sporozoite-induced malaria infection in mice, monkeys, and humans (Nussenzweig et al., 1967; Clyde et al., 1973a,b, 1975; Rieckmann et al., 1974, 1979; Gwadz et al., 1979). In the human studies, mosquitoes infected with malaria sporozoites were exposed to x-rays, and the resulting radiation-attenuated sporozoites were introduced into human volunteers during the mosquitoes ' blood meal.
Immunization with radiation-attenuated sporozoites induces protection unlike that found after natural infection. Naturally acquired immunity seems to be directed primarily against the erythrocytic stages of the parasite, so that infection per se is not prevented, but people are protected from severe disease and death. Individuals who have lived for 20 or more years in endemic regions still become infected, although they may have few or no symptoms (Hoffman et al., 1987). In contrast, volunteers immunized with irradiated sporozoites do not develop any detectable blood-stage infection. The differences may be due in part to the fact that even in areas of the highest malaria transmission, naturally exposed individuals are bitten by relatively few infective mosquitoes (fewer than 50 per month) (Beier et al., 1990). Thus they may not receive sufficient stimulation by sporozoite antigens to induce the protective immune responses achieved by repeated exposure to many hundreds of irradiated, infected mosquitoes over a few weeks or months (Clyde et al., 1973a,b, 1975; Rieckmann et al., 1974, 1979).
Vaccinating people with irradiated sporozoites cannot be routinely done. No culture system capable of producing large numbers of sporozoites is available or perhaps even feasible. Dissecting sporozoites from infected mosquitoes is far too labor intensive, and exposing people to mosquitoes containing irradiated sporozoites is not a tenable strategy. Given these limitations, the only hope for a pre-erythrocytic vaccine lies in the construction of a subunit vaccine, and researchers have been focusing on this objective. Identifying the mechanisms of protective immunity and the parasite antigens against which these protective immune responses are directed is a prerequisite to the development of this type of vaccine. Both antibodies and cellular immune responses contribute to this protection.
Antibody-Mediated Immunity Mice and humans immunized with irradiated sporozoites develop antibodies directed against sporozoites. When administered to animals, some of these antisporozoite antibodies can protect against sporozoite-induced malaria infection (Potocnjak et al., 1980; Egan et al., 1987; Charoenvit et al., 1991a,b). Protective antisporozoite antibodies generally react with only a single species of malaria parasite and thus confer protection only against that species. This is similar to the species-specific immunity induced by immunization with irradiated sporozoites (Clyde et al., 1975).
The mechanisms by which antibodies confer protection are not certain. Antibodies can prevent the invasion of sporozoites into liver cells in culture (Hollingdale et al., 1984; Mazier et al., 1986), and it is likely these antibodies inhibit parasite binding to or invasion of liver cells. Antibodies appear to have other damaging effects on sporozoites, since those that successfully invade liver cells in the presence of antisporozoite antibodies often do not develop normally and fail to release merozoites that can initiate a blood-stage infection (Mazier et al., 1986). Conceivably, antibodies might also kill sporozoites directly or make them more susceptible to phagocytosis.
Cell-Mediated Immunity Cell-mediated immunity is a broad classification that includes all immune responses that involve antigen-primed white blood cells (lymphocytes) or their products, other than antibodies. These are produced by B lymphocytes (so called because their maturation occurs in the bone marrow). The site on an antigen recognized by an antibody is known as a B-cell epitope. The cellular portion of the immune response includes T lymphocytes (so called because their maturation occurs in the thymus), natural killer cells, and monocytes. T lymphocytes have been further subdivided into helper cells (which enhance the production of antibody or stimulate other lymphocytes), suppressor cells (which suppress the production of antibody or inhibit other lymphocytes), and cytotoxic T lymphocytes (which directly kill other cells, including malaria-infected cells). The site on an antigen recognized by a T cell is known as a T-cell epitope.
Both lymphocytes and monocytes secrete proteins, called cytokines, that affect the function of other cells. Some of these cytokines regulate the function of lymphocytes. Other cytokines act to destroy cells, including those that contain infectious agents such as malaria parasites.
Cell-mediated immunity is an important part of the protection induced by immunization with irradiated sporozoites. For example, mice that are unable to produce antibodies can be successfully immunized with irradiated sporozoites (Chen et al., 1977). In addition, administration of immune lymphocytes to normal mice can protect them from sporozoite-induced malaria infection in the absence of anti-sporozoite antibodies (Egan et al., 1987). Experimental subunit sporozoite vaccines that elicit cell-mediated immunity without inducing antisporozoite antibodies also can protect mice against sporozoite-induced malaria (Sadoff et al., 1988). These studies suggest the presence of protective cell-mediated immune responses.
There are a number of mechanisms by which cell-mediated immunity could protect against sporozoite-induced malaria. Cytokines such as gammainterferon may play a role. Gamma-interferon inhibits the development of malaria parasites in cultured liver cells (Ferreira et al., 1986; Mellouk et al., 1987). Treatment with agents that induce interferon production (Jahiel,
1968a,b), or administration of purified gamma-interferon (Maheshwari et al., 1986; Schofield et al., 1987a) will protect animals from sporozoite-induced malaria.
Cytotoxic T lymphocytes are also important. In some mouse strains, these cells appear to be the principal mechanism of protective immunity, since treatment of immunized mice with antibodies that destroy cytotoxic T lymphocytes renders them susceptible to sporozoite-induced malaria (Schofield et al., 1987b; Weiss et al., 1988). Some cytotoxic T lymphocytes recognize malaria-infected liver cells (Hoffman et al., 1989b; Weiss et al., 1990); administration of these cells can protect mice from sporozoite-induced malaria infection (Romero et al., 1989; Tsuji et al., 1990).
A general feature of T lymphocytes is that they do not recognize whole antigens directly. Instead, they recognize fragments of antigens presented as a complex with certain host proteins (the major histocompatibility complex, or MHC, proteins) on the surface of cells. Thus, cell-mediated immune responses will not be directed against extracellular sporozoites in the circulation, but will instead be directed against malaria-infected liver cells (Hoffman et al., 1989b). Because sporozoites invade liver cells within a few minutes or hours but parasite maturation within liver cells takes many days, protective cell-mediated immune responses directed against infected liver cells may be more effective than antisporozoite antibodies.
Targets of Pre-Erythrocytic Immunity
In both humans and laboratory animals, immunity induced by irradiated sporozoites is both stage specific and species specific; that is, immunization with sporozoites does not protect against infection with blood-stage parasites, and immunization with P. falciparum sporozoites does not protect against infection with P. vivax (and vice versa). This suggests that the immune response is directed against species-specific antigens on the sporozoite or the host' s infected liver cells. Significantly, the protection is not strain specific; immunization with one P. falciparum isolate elicits protective immunity against isolates from other regions of the world (Clyde et al., 1973b).
Circumsporozoite Protein For a number of reasons, initial efforts to develop a pre-erythrocytic vaccine have focused heavily on one protein found on the surface of the sporozoite, the circumsporozoite (CS) protein. The CS protein is a target of both protective antibody and cell-mediated immune responses. The gene encoding the CS protein was one of the first malaria genes to be cloned by using molecular biology techniques, and CS genes have been cloned from a large number of human and animal malaria
parasites (Chulay, 1989), which facilitates the testing of vaccines in various animal model systems.
The CS proteins of all malaria parasites studied thus far are similar in size and overall structure but vary considerably in specific composition. All have a central region consisting of tandem repeats of species-specific amino acids (Dame et al., 1984). After immunization with irradiated sporozoites, most of the antibodies that develop are directed against the repetitive region of the CS protein (Zavala et al., 1983). Antibodies directed against the CS repetitive region inhibit sporozoite invasion into cultured liver cells (Mazier et al., 1986), and administration of such antibodies can completely protect against sporozoite-induced malaria in mouse and monkey model systems (Potocnjak et al., 1980; Egan et al., 1987; Charoenvit et al., 1991a,b). In P. falciparum, the repetitive region contains a series of four amino acids (asparagine-alanine-asparagine-proline) that has been detected in all isolates analyzed (Zavala et al., 1985). Thus, protective immunity directed against the repetitive region of P. falciparum would be expected to be species specific but not isolate specific.
Antibodies against non-repetitive regions of the CS protein have also been shown to inhibit sporozoite invasion into cultured liver cells (Aley et al., 1986; D. M. Gordon, Department of Immunology, Walter Reed Army Institute of Research, personal communication, 1990).
The CS protein is also a target of cell-mediated immunity. Mice and humans immunized with irradiated sporozoites develop cytotoxic T lymphocytes directed against portions of the CS protein (Kumar et al., 1988; Romero et al., 1989; Weiss et al., 1990; Malik et al., 1991). Such cytotoxic T lymphocytes can destroy malaria-infected liver cells in culture (Weiss et al., 1990), and administration of cloned T lymphocytes specific for portions of the CS protein can protect mice against sporozoite-induced malaria (Romero et al., 1989; Del Giudice et al., 1990).
The CS protein also contains epitopes recognized by helper T lymphocytes. These T helper epitopes are present in both the repetitive and flanking non-repetitive regions of the CS protein (Good et al., 1987).
Sporozoite Surface Protein 2 A second sporozoite surface protein (SSP2) that is a target of protective immunity has recently been identified in P. yoelii (Charoenvit et al., 1987; Hedstrom et al., 1990). Antibodies against SSP2 partially inhibit sporozoite invasion into liver cells in culture (S. Mellouk, unpublished), and administration of a cloned T lymphocyte that recognizes SSP2 can protect mice against sporozoite-induced malaria (S. Khusmith, unpublished). Immunization of mice with either a CS protein vaccine alone or an SSP2 vaccine alone can protect a proportion of mice against sporozoite-induced malaria, while concurrent immunization with both vaccines protects all mice (Khusmith et al., 1991). This synergistic effect emphasizes one of the advantages of including multiple antigens from a single parasite stage in a multicomponent malaria vaccine.
Other Sporozoite Proteins Antibodies directed against other sporozoite antigens can inhibit sporozoite invasion into liver cells in culture, and when administered to mice can protect them from sporozoite-induced malaria (Hollingdale et al., 1990). Administration of a cloned T lymphocyte that recognizes another sporozoite antigen, that is also expressed by infected erythrocytes, can also protect mice against sporozoite-induced malaria (Tsuji et al., 1990).
Liver-Stage Antigens CS protein and other sporozoite antigens are expressed in malaria-infected liver cells. There are other antigens not found on sporozoites that are first produced by parasites developing within liver cells (Guerin-Marchand et al., 1987; Hollingdale et al., 1990). It is likely that some of these liver-stage antigens contain epitopes that are targets for protective cell-mediated immune responses.
Impediments to Pre-Erythrocytic Vaccine Development
Production of Antigen Sporozoites, like other stages of malaria parasites, cannot be produced in sufficient quantity or purity to be used to immunize humans. Malaria vaccine development has therefore relied on subunit vaccines. A subunit vaccine is generally constructed in three parts: the target of the desired protective immune response (e.g., B-cell epitopes as targets for antibodies and T-cell epitopes as targets for cytotoxic T lymphocytes); carrier peptide(s) to stimulate helper T lymphocytes; and an adjuvant or other delivery system to improve the magnitude and quality of the immune response.
The first malaria sporozoite vaccines tested in humans contained the repetitive B-cell epitope of the CS protein plus T-cell epitopes from nonmalaria proteins and were constructed by using recombinant DNA technology or synthetic peptide chemistry (Ballou et al., 1987; Herrington et al., 1987). Other approaches have utilized entirely synthetic vaccines consisting of B- and T-cell epitopes from the CS protein (Tam et al., 1990) or malaria B-cell epitopes coupled to selected proteins or peptides that have helper but not suppressor epitopes. A peptide from tetanus toxoid with these latter properties has been described (Etlinger et al., 1990). In mice primed with bacillus Calmette-Guerin (BCG), a live, avirulent strain of tuberculosis bacterium, immunization with a vaccine consisting of the B-cell epitope of the P. falciparum CS protein coupled to a purified tuberculosis protein produced high titers of antibodies to sporozoites without the use of any other adjuvant (Lussow et al., 1990). Given the widespread sensitization of humans to tuberculosis proteins and BCG, this vaccine, or a recombinant BCG expressing the CS protein, might be effective.
Recombinant live attenuated vaccines might also be used. For example,
insertion of the CS protein gene into attenuated strains of Salmonella creates a vaccine that can protect mice from sporozoite-induced malaria (Sadoff et al., 1988; Aggarwal et al., 1990). Although it is relatively easy to produce vaccines for animal immunization studies, production of subunit vaccine antigens of sufficient purity for trials in human volunteers is more difficult and expensive. Research laboratories studying malaria immunity are not equipped to produce these high-quality vaccines, and collaboration with pharmaceutical or biotechnology companies is generally necessary.
Induction of the Appropriate Immune Response
ANTIBODIES Administration of monoclonal antibodies directed against specific sporozoite B-cell epitopes can protect animals against sporozoite-induced malaria. Inducing high levels of antibody is thus one objective of immunization with sporozoite vaccines. Early subunit vaccines conferred complete protection from sporozoite-induced malaria in a small number of immunized volunteers who were experimentally exposed to the bites of infected mosquitoes, and they delayed the onset of blood-stage infection in other volunteers who had antibodies (Ballou et al., 1987; Herrington et al., 1987). Overall, however, these vaccines induced relatively low levels of antibody (Chulay, 1989). These antibody levels were lower than those to the same vaccine in mice and rabbits. They were also lower than the highest antibody levels induced by natural exposure to the bites of sporozoite-infected mosquitoes (Hoffman et al., 1987), and such levels of antibody are not protective.
One way to increase the level of antibody induced by sporozoite vaccines is to change the carrier protein and the adjuvant. Early results suggest this approach can increase the level of antibody 10-fold (Rickman et al., 1991).
The quantity of antibody is not the only determinant of protection, however. High levels of antisporozoite antibodies were achieved after immunization with the P. falciparum repetitive B-cell epitope coupled to a bacterial protein, but only one of eight volunteers was protected against malaria, and that individual did not have one of the highest levels of antibody (L. Fries, Associate Professor of International Health, Center for Immunization Research, Johns Hopkins School of Hygiene and Public Health, personal communication, 1990). The specificity of the antibody response is also important. For example, monkeys can be protected from sporozoite-induced P. vivax malaria by administration of a monoclonal antibody directed against the CS protein (Charoenvit et al., 1991b). This monoclonal antibody recognizes a four-amino-acid B-cell epitope contained within the nine amino acids of the repetitive portion of the CS protein (Charoenvit et al., 1991b). A recombinant P. vivax sporozoite vaccine containing all
nine amino acids of the CS repeat section that contained only the repetitive amino acids of the CS protein induced high levels of antisporozoite antibodies in monkeys and humans (Collins et al., 1989; Gordon et al., 1990), but these antibodies were not directed against the four-amino-acid B-cell epitope recognized by the protective monoclonal antibody (Charoenvit et al., 1991b).
Protection does not appear to be a function of the specific immunoglobulin G (IgG) subclass of antibodies, since monoclonal antibodies of the IgG1, IgG2b, and IgG3 subclasses, as well as Fab fragments (antibodies lacking their subclass-specific portion), have all been shown to transfer protection passively in mice (Potocnjak et al., 1980; Egan et al., 1987; Charoenvit et al., 1991a,b). Protection also does not appear to be explained by differences between the structure of the native sporozoite protein and the synthetic and recombinant peptide subunit vaccines, since monoclonal antibodies produced by immunization with short peptides can also confer protection when administered to animals.
Immunization undoubtedly produces polyclonal antibodies of varying affinities and specificities. To achieve consistent antibody-mediated protection, a vaccine may have to focus the immune response, or dramatically increase the overall production of antibody, to achieve appropriate concentrations of the “correct” antibodies.
PASSIVE IMMUNIZATION Because passive transfer of monoclonal antibodies is so effective in protecting against sporozoite-induced malaria in animals, some researchers are working to develop human monoclonal antibodies against the repeat regions of the human malaria CS protein. These antibodies could be used to passively immunize short-term visitors to malarious areas in the same way that gamma globulin is used to prevent hepatitis A.
CELL-MEDIATED IMMUNE RESPONSE Little is known about how to induce protective cell-mediated immune responses with subunit vaccines. Cytotoxic T lymphocytes generally are not induced by immunization with standard preparations of inactivated microbial antigens. Cytolytic T lymphocytes can be induced by immunization with recombinant live attenuated vaccines, such as those for salmonella, BCG, and vaccinia (smallpox vaccine). Work is in progress to construct recombinant live attenuated vaccines that express malaria genes and that might induce protective cell-mediated immune responses in humans.
Cytotoxic T lymphocytes can also be induced by immunization with antigens contained within liposomes (small lipid-bound vesicles that can interact with cells of the immune system), immunostimulatory complexes (particles formed by antigen complexed with the detergent saponin) (Takahashi et al., 1990), and peptides containing a cytotoxic T-lymphocyte epitope
coupled to a lipid structure (Deres et al., 1989). These offer additional possible approaches to induction of protective cell-mediated immune responses.
Antigenic Diversity Most malaria antigens appear to have significant antigenic diversity. Much of this diversity may be an adaptive response of the parasite to host immunity, and it is theoretically possible that an initially effective malaria vaccine might select for parasite variants, leading to vaccine-resistant strains.
For example, there are at least two major variants of the repetitive region of the CS protein of P. vivax, and antibodies that recognize one variant do not react with the other (and vice versa) (Rosenberg et al., 1989; Wirtz et al., 1990). Extensive variation in the CS protein repetitive region occurs in the monkey malaria parasites P. knowlesi and P. cynomolgi (Sharma et al., 1985; Galinski et al., 1987). For vaccines intended to induce antibodies directed against the CS repetitive epitope, variability in the repetitive region would complicate vaccine formulation, since a useful vaccine must protect against all variants. For P. falciparum, variation in the repeat region of the CS protein has thus far been limited to minor changes in the numbers of repeating units and, occasionally, changes in a few of the individual repetitive epitopes. All P. falciparum isolates obtained from different parts of the world are recognized by monoclonal antibodies directed against the predominant repetitive epitope (Zavala et al., 1985).
Variability in T-cell epitopes may also be important. For example, the DNA sequence of the CS protein gene from several different isolates has been determined. It is highly conserved, and the variations that do occur are clustered within regions that code for T-cell epitopes (Good et al., 1987; Kumar et al., 1988; Lockyer and Schwarz, 1987). Variation in helper T-cell epitopes may potentially limit the ability of sporozoites to boost the antibody response to CS protein B-cell epitopes, and variations in cytotoxic T-lymphocyte epitopes may allow liver-stage parasites to evade killing by cytotoxic T lymphocytes.
Genetic Restriction of the Immune Response T lymphocytes recognize fragments of antigens that are presented as a complex with host MHC proteins on the surface of cells (see above). The antigen fragments (T-cell epitopes) are formed by the action of cellular enzymes that digest the antigen into small pieces of about 8 to 12 amino acids.
The interaction of T-cell epitopes with MHC proteins is highly specific. Changing a single amino acid in either the T-cell epitope or the region of the MHC protein that binds to the T-cell epitope can completely prevent the interaction. There is tremendous variability among individuals in the genetically determined amino acid sequence of the region of the MHC
protein that binds to T-cell epitopes. This means that only the T lymphocytes of some individuals (those with suitable MHC proteins) can recognize a given T-cell epitope. Genetic restriction is most easily studied in inbred mouse strains that have MHC proteins that are identical for all individuals of that strain but different from the MHC proteins of other strains. Most T-cell epitopes on malaria antigens appear to be genetically restricted (Del Giudice et al., 1986; Good et al., 1986, 1987, 1988a,b,c; Good, 1988; Hoffman et al., 1989a). That is, only mice of a few strains can recognize these epitopes. The human cell-mediated immune response to malaria antigens also appears to be genetically restricted (Good, 1988; Good et al., 1988d; Hoffman et al., 1989c; de Groot et al., 1989).
If only a minority of individuals can mount effective immune responses against protective T-cell epitopes after immunization, a vaccine would have to include multiple T epitopes, perhaps from different target antigens, to increase the probability that at least one T-cell epitope was recognized by almost every individual within a population. Indeed, the apparently universal ability of live attenuated sporozoite vaccines to induce protective immunity may result in part from the presence of multiple T-cell epitopes on multiple different target antigens. Although knowledge in this area is limited, it is encouraging that three of four volunteers immunized with irradiated sporozoites produced cytotoxic T lymphocytes against a defined T-cell epitope in the P. falciparum CS protein (Malik et al., 1991). The importance of genetic restriction to malaria antigens in humans remains to be determined.
Assays Predictive of Protection One of the major obstacles to malaria vaccine development is the lack of laboratory assays that correlate with protective immunity (Hoffman et al., 1987). The only certain way to test vaccine efficacy is to immunize volunteers and determine whether they are protected after exposure to malaria-infected mosquitoes. Development of laboratory assays that predict protective immunity would be a major advancement.
Length of Protection Nonimmune visitors to endemic areas may need a vaccine that protects them for only several weeks or months. In contrast, long-term residents of endemic regions require a vaccine that provides protection for years.
Immunization with live attenuated sporozoites induces long-lasting protective immunity in mice, but in the few humans tested protection disappears after a few months (Clyde et al., 1973b, 1975; Rieckmann et al., 1979). The early experience with malaria subunit vaccines based on the P. falciparum CS protein and tested in humans indicates that antibody levels generally decline quickly. Approaches that may overcome this problem
include the use of adjuvants that increase the antibody response, so that protective levels of antibody are maintained for longer periods; the addition of helper T-cell epitopes from malaria antigens to boost antibody levels after natural exposure to sporozoites; and the use of new vaccine formulations that provide prolonged release of antigen, thereby providing their own booster doses over time. It must be acknowledged that such formulations have not been developed for any vaccine and represent a considerable technological challenge.
Asexual Blood-Stage Vaccines
An erythrocytic (blood-stage) or merozoite vaccine is intended to control parasite numbers after their release from the liver, thus preventing or reducing malaria-related morbidity and mortality. Even partial immunity to the asexual blood-stage parasites could be important, since disease severity is related to the level of parasitemia. This can be visualized by considering a hypothetical “typical” P. falciparum infection. In a nonimmune individual (Figure 9-2A), no blood-stage parasites would be present until about day 5, and clinical illness would not appear until several days later, after one or more cycles of parasite multiplication within red blood cells. Unless the individual were treated or rapidly developed a protective immune response, the parasitemia would increase exponentially to reach life-threatening levels within about a week, and death would follow shortly thereafter.
In an individual with immunity directed against only the pre-erythrocytic stages of the parasite (Figure 9-2B), 80 percent, 90 percent, or 95 percent inhibition of parasite multiplication would delay the onset of clinical illness by two to four days, but would have no effect on the subsequent exponential increase in parasitemia and progression to life-threatening infection.
In an individual with immunity directed against only blood-stage parasites (Figure 9-2C), 80 percent inhibition of parasite multiplication would delay the onset of clinical illness by about one week and markedly prolong the time until development of life-threatening infection. Assuming a 10-fold multiplication rate in a nonimmune individual, 90 percent inhibition of parasite multiplication would result in no net increase in parasitemia, and thus theoretically could prevent clinical illness without resolving the infection. With 95 percent inhibition, there would be a progressive decrease in parasitemia and eventual resolution of infection.
Thus, many believe that the most important and realistic goal for malaria vaccine development is to identify antigens and immune responses that protect adults and children living in endemic areas from becoming severely ill or dying of the disease. This immune response seems primarily to target the erythrocytic stage of the parasite.
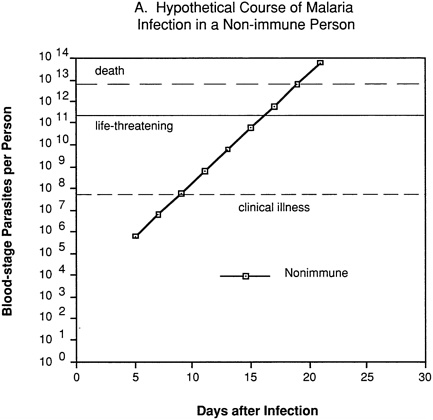
FIGURE 9-2 Hypothetical course of Plasmodium falciparum infection in a nonimmune individual (A) and the effect of various levels of host immunity to sporozoites (B) or to blood-stage parasites (C). Note: Graphs were generated assuming (1) a mosquito injects 20 sporozoites, (2) each sporozoite yields a liver-stage parasite that gives rise to 30,000 merozoites that infect red blood cells, (3) the individual has 5 million red blood cells per microliter of blood and 6 liters total blood volume, (4) the blood-stage parasites multiply 10-fold every 48 hours, (5) clinical illness develops when parasitemia reaches about 10 per microliter of blood, (6) life-threatening illness develops when about 1 percent of the red blood cells are parasitized, and (7) death is almost inevitable when more than 20 percent of the red blood cells are parasitized. Based on unpublished calculations supplied by J. D. Chulay (Institute of Medicine Malaria Committee member) and J. D. Haynes (Walter Reed Army Institute of Research, Washington, D.C.)
Mechanisms of Immunity
Antibody Mediated Epidemiologists and clinicians working in malarious areas have long recognized there is an exposure-dependent acquisition of immunity to malaria. Because they benefit from maternal antibodies, infants are less likely than young children to become ill or die from malaria;
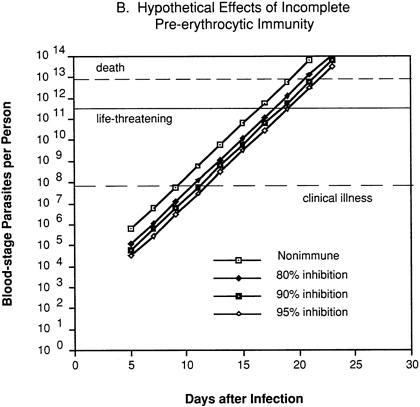
in older children and adults, illness and death caused by malaria decrease with increasing exposure. This immunity is mediated primarily by antibodies against blood-stage parasites, and children with P. falciparum malaria who are treated with antibodies from the serum of hyperimmune adults show a dramatic reduction or even an elimination of parasitemia (Cohen et al., 1961; Bouharoun-Tayoun et al., 1990).
In infancy, these antibodies are transferred across the placenta to the fetus. In older children and adults, they are produced as a result of infection with malaria parasites. This protective immune response is not strain specific, since West African gamma globulin is effective in reducing parasitemia in children with malaria in East Africa (McGregor et al., 1963) and Thailand (Bouharoun-Tayoun et al., 1990).
The mechanisms by which antibodies confer protection from malaria are not completely understood. Antibodies could inhibit parasite invasion of red blood cells by agglutinating merozoites as they are released from schizont-infected erythrocytes, thus rendering them incapable of invasion (Miller et al., 1975;
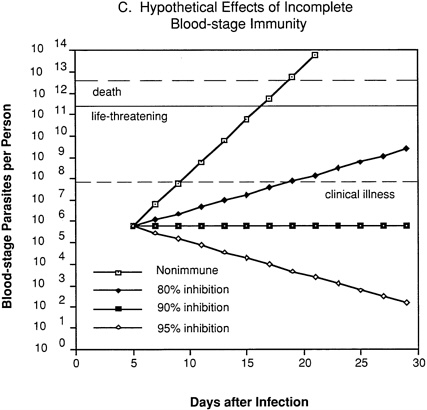
Chulay et al., 1981), by blocking the binding of merozoites to erythrocytes (Thomas et al., 1984; Sim et al., 1990), or by interfering with the function of parasite proteins, such as proteases or rhoptry/microneme proteins, that mediate the invasion process (Holder and Freeman, 1984). Antimerozoite antibodies might also conceivably kill the parasites directly or make them more susceptible to phagocytosis (Khusmith and Druihle, 1983).
Antibodies that recognize parasite proteins on the surface of infected erythrocytes could contribute to the destruction of the cells. The process could be accomplished through complement-mediated lysis; by phagocytosis and antibody-dependent cellular inhibition (Bouharoun-Tayoun et al., 1990); by inhibition of the transport of nutrients essential to parasite growth (Cabantchik et al., 1983; Elford et al., 1985); or by inhibition of the attachment of infected erythrocytes to endothelial cells, thus preventing them from obstructing the microcirculation and diverting them to the spleen, where they will be removed from the circulation (David et al., 1983; Udeinya et al., 1983).
Antibodies also could react with parasite antigens, released from infected erythrocytes, that mediate the disease process (Playfair et al., 1990). This could occur through direct inhibition of parasite toxins, or by blockage of the interaction of antigens with host cells (such as macrophages), thereby preventing the release of toxic substances like tumor necrosis factor (TNF) and other cytokines. It is not clear that this response would resolve the infection or completely prevent the pathology resulting from infection.
Cell Mediated Cell-mediated immune responses appear to be important in malarial immunity, at least in animal models, but the mechanisms involved are unclear. Helper and suppressor T lymphocytes are undoubtedly important in modulating antibody levels. Because T lymphocytes recognize antigens on the surface of cells only in conjunction with MHC molecules, and mature human erythrocytes do not express MHC molecules, it is unlikely that T cells could recognize infected erythrocytes directly.
Some parasite antigens are secreted from malaria-infected erythrocytes (Howard et al., 1986) and, when taken up by macrophages or other antigen-presenting cells, may stimulate T-cell secretion of cytokines, some of which are known to be toxic to malaria parasites (Ockenhouse et al., 1984). It is possible that local concentrations of cytokines may be high enough to cause the destruction of the intra-erythrocytic malaria parasites.
Targets of Blood-Stage Immunity
The identification of targets of protective immunity has been based in part on an understanding of the mechanisms of immunity to blood-stage malaria parasites and in part on empirical observations. Several candidate proteins have been identified and are listed in Table 9-1. Some of the criteria used to select candidate vaccine antigens are discussed below.
Merozoite Invasion Interference with merozoite attachment to and invasion of erythrocytes has been a major focus of many research groups. Invasion is a multistep process involving receptor binding, “tight-junction” formation, erythrocyte-membrane invagination, vacuole formation, and closure (Hadley and Miller, 1988; Perkins, 1989). Parasite proteins involved in each of these steps are potential vaccine candidates.
There are at least two erythrocyte receptors to which P. falciparum merozoites bind. One of these is probably a member of a family of sialic acid-rich glycoproteins, the glycophorins (Jungery, 1985), while the other is
TABLE 9-1 Candidate Antigens for Blood-Stage Malaria Vaccines
Antigen |
Synonyms |
Target of Inhibitory Antibodies |
Protection in Primate Trial |
Model Homologue |
ICM |
Sequence Diversitya |
MSA-1 |
PMMSA, PSA, p185, p190 |
+ |
+ |
+ |
+ |
+ |
MSA-2 |
Gymmsa, gp56, 38-45 kDa antigen |
+ |
n.d. |
n.d. |
+ |
+ |
RESA |
Pf155 |
+ |
+ |
+ |
? |
− |
EBA |
+ |
n.d. |
n.d. |
− |
minor |
|
AMA-1 |
Pf83 |
− |
n.d. |
+ |
− |
minor |
SERA |
p113, p126 SERP, Pf140 |
+ |
+ |
n.d. |
+ |
minor |
RAP-1 |
+ |
+ |
n.d. |
− |
n.d. |
|
RAP-2 |
− |
+ |
n.d. |
− |
n.d. |
|
RhopH3 |
+ |
+ |
n.d. |
− |
− |
|
PfHRP-II |
− |
+ |
n.d. |
− |
− |
|
Pf55 |
− |
+ |
n.d. |
− |
n.d. |
|
Pf35 |
− |
+ |
n.n. |
− |
n.d. |
|
GBP |
96-R |
− |
− |
n.d. |
+ |
− |
ABRA |
p101 |
− |
n.d. |
n.d. |
+ |
− |
Exp-1 |
CRA, Ag5.1 |
+ |
+ |
n.d. |
? |
minor |
Aldolase |
− |
− |
n.d. |
− |
n.d. |
|
HSP70-1 |
p75 |
− |
− |
− |
− |
− |
a Single amino acid changes may cause changes in antigenicity for B and T-cells (see Chapter 6). Abbreviations used in this table: 96-R, 96 kDa thermostable protein; ABRA, acidic base repeat antigen; Ag5.1, antigen 5.1; AMA-1, apical merozoite antigen 1; CRA, circumsporozoite protein related antigen; Exp-1, exported protein 1; EBA, erythrocyte binding antigen; gp56, glycoprotein with an apparent molecular mass of 56 kDa; GBP, glycophorin binding protein; Gymmsa, glycosylated and major merozoite surface antigen; HSP70-l, 70-kDa heat shock protein 1; ICM = Immune clustered merozoites; kDa, kilodaltons; MSA-1, merozoite surface antigen 1; MSA-2, merozoite surface antigen 2; n.d., not determined; p75, p101, etc., proteins with an apparent molecular mass of 75 kDa, 101 kDa, etc.,; Pf83, Pf155, etc., P. falciparum protein with an apparent molecular mass of 83 kDa, 155 kDa, etc.; PfHRP-II, P. falciparum histidine rich protein II; PMMSA, precursor to the major merozoite surface antigen; PSA, polymorphic schizont antigen; RESA, ring-infected surface antigen; RAP-l, rhoptry associated protein 1; RAP-2, rhoptry associated protein 2; RhopH3, rhoptry high molecular weight complex protein 3; SERA, serine rich antigen; SERP, serine rich protein. |
unrelated to the glycophorins (Mitchell et al., 1986). In addition, it has been proposed that the parasite itself releases proteins, called erythrocyte-binding antigens, that act as a bridge between the merozoite and the erythrocyte. At least one of these has been identified (Camus and Hadley, 1985) and characterized (Orlandi et al., 1990; Sim et al., 1990).
For P. vivax, the Duffy blood group antigen is the major erythrocyte receptor (Miller et al., 1976). The parasite antigens that bind to these receptors are potential vaccine candidates. Where merozoites of a single
Plasmodium species can invade by binding to one of several erythrocyte receptors, a multivalent vaccine will be required to completely inhibit merozoite invasion of red blood cells.
Rhoptries and micronemes are organelles located near the anterior end of the merozoite. Release of the contents of these organelles is essential to erythrocyte invasion, and there is evidence this process is susceptible to antibody attack (Holder and Freeman, 1984).
Antibodies from immune individuals can bind to the surface of merozoites as they are released from schizont-infected erythrocytes, forming large merozoite clusters incapable of invading erythrocytes (Chulay et al., 1981). Antibodies dissociated from these immune complexes have been used to identify the antigens involved (Chulay et al., 1987; Lyon et al., 1989). These include integral membrane proteins present on the merozoite surface as well as proteins that are only loosely associated with the merozoite surface.
Erythrocyte Surface Antigens Antigens on the surface of infected erythrocytes are a particularly attractive target for vaccine intervention because of the long period during which they are exposed to the immune system. In P. falciparum, some of these antigens mediate the adherence of infected erythrocytcs to endothelium, which causes mature-stage erythrocytic parasites to become sequestered in blood capillaries, where they are able to avoid destruction by the spleen (Howard et al., 1990).
Erythrocytes parasitized by mature P. falciparum in vitro can adhere to at least three different host endothelial cell molecules: CD-36, thrombospondin, and ICAM-1 (CD-54) (Howard and Gilladoga, 1989; Chulay and Ockenhouse, 1990). Parasitized erythrocytes can also adhere to (rosette with) normal erythrocytes, another type of cell-to-cell interaction (Udomsangpetch et al., 1989). The parasite molecules responsible for adherence have not been completely identified, but it appears that at least one is a high molecular weight (250 to 300 kilodaltons) variable antigen called P. falciparum erythrocyte membrane protein 1 (Leech et al., 1984; Howard et al., 1990). This protein has multiple distinct serotypes and may also undergo true antigenic variation. There is evidence that partially immune adults develop antibodies able to recognize epitopes that are conserved among all serotypes (Marsh and Howard, 1986).
Antigens Crucial to Parasite Growth Monoclonal or polyclonal antibodies have been added to in vitro culture systems and screened to identify those that inhibit parasite growth (Perrin et al., 1981; Saul et al., 1984, 1985; Schofield et al., 1986; Lew et al., 1989). Protection of animals by passive transfer of these antibodies can confirm their value. The antigens recognized by such inhibitory antibodies are of obvious interest.
Antigens That Induce Protective Immunity in Animal Models A number of animal malarias, such as those caused by P. chabaudi and P. yoelii in mice, P. lophurae in ducklings, and P. fragile and P. knowlesi in rhesus monkeys, have been used to identify antigens that can induce protective immunity. The hope is that homologous molecules from human malaria parasite species will make good vaccine candidates. Experimental immunization with merozoite surface antigen 1 (MSA-1) in various animal models supports this hope (Holder and Freeman, 1981; Schmidt-Ullrich et al., 1983; Patarroyo et al., 1987; Siddiqui et al., 1987).
P. falciparum has been adapted to grow in aotus and saimiri monkeys, and partially purified parasite antigens that confer protection have been identified (Patarroyo et al., 1987). A number of blood-stage proteins were purified and tested individually for protective efficacy in aotus monkeys. The sequence of proteins that induced a degree of protection was determined, and synthetic peptides were made and used to immunize aotus monkeys. A combination of peptides from three proteins induced a protective immune response that resulted in survival from an otherwise lethal infection, often with little or no parasitemia.
Pathogenic Immune Factors Efforts to produce vaccines that prevent the most severe symptoms and signs of malaria disease represent an exciting new approach to malaria vaccine development. The impetus for research in this area comes from the observation that older children and repeatedly infected adults in endemic areas may harbor significant parasite concentrations without showing symptoms of disease. This tolerance is lost fairly quickly after exposure to malaria ceases. There is also similarity between the symptoms of malaria and the symptoms produced in nonmalarious individuals by treatment with TNF, interferons, and some of the interleukins. There is also a correlation between the severity of symptoms in severe malaria and the level of circulating TNF (see Chapter 4).
Soluble exoantigens of bloodstage P. yoelii, P. berghei, and P. falciparum, probably released at the time of schizont rupture, induce the secretion of TNF from mouse peritoneal macrophages and human peripheral blood lymphocytes in vitro (Bate et al., 1988; Taverne et al., 1990). These exoantigens, which appear to be phospholipids, also induce TNF production in vivo and can kill mice made sensitive to it (Bate et al., 1989). When exoantigens are used as immunogens, they induce a T-cell-independent IgM antibody response (Bate et al., 1990) that protects mice from dying from what would ordinarily be a lethal malaria infection (Playfair et al., 1990). This antibody response also blocks the ability of exoantigens to induce TNF production in vitro. Exoantigens appear to be conserved across animal species, since antisera against rodent malaria exoantigens recognize human exoantigens, and vice versa.
Preclinical and Clinical Trials
To date, the only reported human trials of asexual-stage malaria vaccines have been performed by Dr. Patarroyo and his colleagues in Colombia (Patarroyo et al., 1988). The vaccines were synthetic peptides based on studies in aotus monkeys (Patarroyo et al., 1987). All immunized subjects became clinically ill, but the infection in three of five volunteers receiving one of the vaccines was controlled without treatment, while all nonimmunized control subjects required treatment. This trial called for treatment only for individuals with more than 0.5 percent of their erythrocytes infected with parasites. No efficacy would have been demonstrated in this trial if a more conservative threshold for starting treatment had been used (see below). The mechanism of immunity, and the role of immune responses against the different antigens included in the vaccine, have not been established. Results of more recent clinical trials with this vaccine are not available for analysis.
Impediments to Asexual Blood-Stage Vaccine Development
Many of the same obstacles to the development of pre-erythrocytic vaccines are relevant to asexual blood-stage vaccines. Several additional impediments are worth mentioning.
Animal Models Animal models for vaccine challenge with P. falciparum and P. vivax are not optimal. Parasites often require extensive adaptation before they will grow in aotus monkeys, and splenectomy is required to get reproducible infections in saimiri monkeys. Further, there are only a few parasite isolates adapted to monkeys, making testing of antigenically diverse vaccine antigens difficult. Variation in the course of infection in control-group monkeys has made interpreting results difficult for vaccines that produce only partial protection. Despite its shortcomings, the aotus monkey model system remains the best available for simulating human P. falciparum infection.
Clinical Trials The outcome of a sporozoite vaccine trial is relatively simple to measure, since any appearance of parasites in the bloodstream signals failure. In contrast, an asexual-stage vaccine that controls parasite multiplication and protects against morbidity and mortality would be considered useful, even if it allowed moderate levels of parasitemia. Because the clinical effects of parasitemia vary with the immune status of the individual, determining when such a vaccine is effective is problematic. Trials performed in nonimmune volunteers, for example, may have to be terminated when only 0.05 percent of erythrocytes are infected. Parasitemia
may increase 10- or 20-fold during the next cycle of asexual development, and parasitemias of about 1 percent may be associated with severe disease and some risk of death, despite appropriate treatment. Vaccines that control infection only after higher levels of parasitemia are reached would not be recognized in such a trial, even though such vaccines might be beneficial in partially immune individuals who were tolerant to modest levels of parasitemia. Candidate vaccine antigens may therefore need to be tested twice before efficacy is known: once in nonimmune volunteers and a second time in individuals living in an endemic area. This adds a further layer of logistical complexity to the vaccine evaluation process.
Antigen Complexity Malaria infection stimulates immune responses to a vast array of asexual blood-stage antigens. It is difficult to predict a priori whether a particular antigen will stimulate a protective immune response. Many of these antigens show considerable sequence variability, and some appear to undergo antigenic variation. In addition, many are too large to be expressed efficiently in recombinant vector systems, and because of their size they are difficult to analyze with use of synthetic peptides. A detailed look at one blood-stage antigen will illustrate many of the problems that are faced.
Merozoite Surface Antigen 1: A Case Study in Vaccine Development
MSA-1 is a glycoprotein (Holder, 1988) anchored by myristic acid to the surface of the schizont (Haldar et al., 1985). When the schizont ruptures, the antigen is processed into a number of discrete products residing on the surface of the merozoite, most of which disappear at the time the merozoite invades red blood cells. Only the carboxy-terminus of MSA-1 carries over into the ring-form trophozoite stage of the parasite (see Figure 9-1). MSA-1 from different P. falciparum isolates varies in size from 180 to 220 kilodaltons, and homologues are present in all Plasmodia species studied. The protein is encoded by a single exon on parasite chromosome 9 (Holder et al., 1985; Mackay et al., 1985; Tanabe et al., 1987; Peterson et al., 1988a).
Monoclonal antibody-binding studies have demonstrated that MSA-1 from different strains contains both conserved and variable regions (McBride et al., 1985). Gene-sequencing studies have confirmed this, and results from several groups have allowed the gene coding region to be subdivided into conserved, semivariable, and variable blocks (Tanabe et al., 1987; Peterson et al., 1988a,b). The variable blocks are generally one of two types (alleles), raising the possibility that a limited number of antigens could be used to protect against all variants of MSA-1. In addition to the two alleles, there are isolate-specific mutations that might allow the parasite to evade allele-specific immunity.
There is considerable evidence that MSA-1 is the target of a protective immune response. Two groups have reported that human monoclonal antibodies against P. falciparum MSA-1 inhibit growth of the parasite in vitro (Brown, 1986; Schmidt-Ullrich et al., 1986). Passive transfer experiments using anti-MSA-1 monoclonal antibodies have demonstrated protection against P. yoelii and P. chabaudi infection in mice (Boyle et al., 1982; Majarian et al., 1984; Burns et al., 1989). These monoclonal antibodies recognize epitopes in the carboxy-terminal half of MSA-1, the portion of the antigen that carries over into the trophozoite stage of the parasite (Burns et al., 1989; Lew et al., 1989).
Active immunization studies with purified MSA-1 have shown protection against P. yoelii in mice (Holder and Freeman, 1981), P. knowlesi in saimiri monkeys (Schmidt-Ullrich et al., 1983), and P. falciparum in saimiri and aotus monkeys (Hall et al., 1984; Cheung et al., 1985; Siddiqui et al., 1987). Immunization with synthetic peptide fragments of MSA-1 has demonstrated some efficacy in saimiri and aotus monkeys (Cheung et al., 1986; Patarroyo et al., 1987). Finally, MSA-1 has been used as part of a combination-peptide antigen in the human trial reported by Patarroyo et al. (1988). Of some concern, however, is the report that mice immunized with MSA-1 and challenged with a different P. chabaudi strain were unprotected (Brown et al., 1985). The full meaning of such results can be determined only after the structure of MSA-1 in different parasite strains is known.
Since immunization with MSA-1 provides at least partial protection against malaria, it is likely that immunogens derived from MSA-1 will be included in a successful multivalent malaria vaccine. Efforts to express MSA-1 by recombinant DNA techniques have so far failed, due in part to the difficulty in obtaining the correct conformational structure of the protein. Recent research using baculovirus vectors may have solved this problem (Murphy et al., 1990), and it is likely that recombinant MSA-1 fragments will soon be tested in humans.
Transmission-Blocking Vaccines
A vaccine that induces an immune response to the sexual stages of the malaria parasite will not necessarily protect against infection with sporozoite or blood-stage parasites. It may, however, have a dramatic effect on reducing malaria transmission by slowing or halting human-to-mosquito (and thus mosquito-to-human) spread of infectious parasites. A transmission-blocking vaccine can be considered “altruistic, ” as it benefits primarily the uninfected or nonimmune individuals in a community, providing little or no benefit to those actually immunized. (Carter et al., 1988; Kaslow, 1990; Targett et al., 1990).
Mechanisms of Immunity
Antibodies Sera from animals immunized with gametocyte-rich blood develop antibodies that, when mixed with gametocytes before ingestion by mosquitoes, will inhibit parasite development and maturation to oocysts within the mosquito (Carter and Chen, 1976; Gwadz, 1976). Monoclonal antibodies directed against gamete and zygote antigens will also inhibit parasite development in mosquitoes via several mechanisms. Some monoclonal antibodies require serum complement and act before fertilization to lyse gametes (Quakyi et al., 1987). Other monoclonal antibodies are complement independent and act either before fertilization, inhibiting zygote formation (Rener et al., 1983; Vermeulen et al., 1985), or work after fertilization (Vermeulen et al., 1985), inhibiting zygote penetration through the peritrophic membrane (Sieber et al., 1991). It is theoretically possible that antibodies could also inhibit zygote-ookinete transformation, inhibit ookinete motility, or inhibit ookinete penetration at later stages of parasite development.
Cell-Mediated Immunity Administration of immune T lymphocytes can reduce the density of gametocytes in the blood of recipient animals and reduce the ability of mosquitoes to transmit parasites by 95 percent (Harte et al., 1985). Helper and suppressor T lymphocytes are presumably also important for modulating antibody production. There is no evidence that ingestion of immune T cells can inhibit parasite development in the mosquito.
Targets of Transmission-Blocking Immunity
The sexual stage of the parasite life cycle provides several potential targets for immunological attack. As with the asexual stages, parasite antigens on the surface of erythrocytes infected with sexual stages could be targeted. Most of the research on transmission-blocking vaccines has focused on the extracellular sexual stages (gametes, zygotes, and ookinetes) found in the mosquito, in part because it is easy to measure antibodies against them, by feeding mosquitoes on infected blood mixed with antibodies and looking for inhibition of parasite development within the mosquito.
A number of proteins on the surface of sexual stages of malaria parasites have been identified by using monoclonal antibodies that inhibit parasite development in mosquitoes (Carter et al., 1988). These antigens are generally named by the initials of the parasite species (e.g., Pf for P. falciparum), the letter “s” (for sexual), and the approximate molecular weight in kilodaltons of the protein (Kaslow et al., 1988). Pfs230, Pfs48/45, and Pfs40/10 are expressed predominantly by gametes. Pfs25 is expressed predominantly
by zygotes (Kaslow et al., 1988). Analogous targets of transmission-blocking antibodies have been identified for P. vivax (Peiris et al., 1988) and various animal malaria parasites.
Impediments to Transmission-Blocking Vaccine Development
Production of Antigen It has been difficult to clone the genes encoding the antigens that are targets of transmission-blocking immunity. Monoclonal antibodies against these antigens appear to recognize conformational epitopes that are not faithfully reproduced by the bacteria in which recombinant DNA libraries are prepared. The use of polyclonal antisera has resulted in cloning other, nontarget antigen genes. It is difficult to culture amounts of sexual stage parasites sufficient to determine the amino acid sequence, which would allow an alternative approach to antibody-based identification. This has slowed the research pace, but various groups appear to have cloned the genes for most or all of the P. falciparum sexual-stage target antigens. Even when the genes are cloned, however, the fact that protective monoclonal antibodies recognize conformational epitopes suggests that expression of recombinant proteins or synthetic peptides that mimic that conformation may be difficult.
Genetic Restriction of the Immune Response Target antigens of transmission blocking antibodies appear to fall into two categories. For Pfs230, Pfs48/ 45, and Pfs40/10, there is significant genetic restriction of T-cell responses in inbred mice (Good et al., 1988b), and only a minority of people living in malaria-endemic areas develop antibodies against these antigens after repeated malaria infections (Graves et al., 1988; Carter et al., 1989; Quakyi et al., 1989). In addition, antigenic variants of Pfs48/45 have been detected (Graves et al., 1985). If genetic restriction proves to be responsible for the limited human immune response to these antigens, this could have an impact on the development of subunit vaccines.
In contrast, Pfs25 is immunogenic and induces transmission-blocking antibodies in all congenic mouse strains tested (Good et al., 1988b; Kaslow et al., 1991), and antibodies are never found in humans living in endemic regions. Pfs25 also was found to lack significant antigenic diversity (Kaslow et al., 1989).
These data suggest that the first group of antigens has been under selective pressure by the host immune system. The absence of genetic restriction in mice and the lack of antibodies to Pfs25 in people living in endemic areas suggest that Pfs25 may be expressed in abundant amounts only when the parasite is in the mosquito midgut, not while circulating in the host's blood, and thus has not been under immune pressure. In either case, the poor or absent human immune response suggests that we may not
be able to rely on natural boosting after primary immunization with a transmission-blocking vaccine. The use of novel adjuvants, carrier proteins, and slow-release vaccine formulations may overcome this problem.
Enhancement of Infectivity At least with P. vivax and the monkey malaria parasite P. cynomolgi, many naturally infected individuals develop antibodies that inhibit parasite development in mosquitoes (Mendis et al., 1987). Early in the course of infection and very late after infection, when the levels of transmission-blocking antibodies are low, parasite development and transmission may be enhanced (Peiris et al., 1988). Enhancement is also observed with low concentrations of some inhibitory monoclonal antibodies, although not with antibodies to Pfs25 and its homologous antigens in other malaria parasites. Although this may superficially suggest that transmission-blocking vaccines may actually enhance transmission as vaccine-induced antibody levels decline, it seems unlikely that vaccination would exacerbate the enhancement that occurs naturally (and may be an important factor sustaining malaria transmission in some areas).
Cost and Acceptability of an Altruistic Vaccine Because transmission-blocking vaccines will not protect individuals, they would not be used alone in nonimmune visitors to malaria-endemic areas. Consequently, there is even less commercial incentive to develop these vaccines than pre-erythrocytic or blood-stage vaccines. Vaccines that protect populations but not individuals might also have to be safer and have fewer side effects than vaccines that provided protection to the vaccinated individual. It is likely that transmission-blocking vaccines will be used primarily in conjunction with pre-erythrocytic and blood-stage vaccines, in which case they may have the added benefit of reducing the transmission of vaccine-resistant parasite strains.
Antimosquito Vaccines
Of the many methods proposed and tested for controlling mosquito populations, one of the most unusual is the use of antimosquito vaccines. In this approach, humans would be vaccinated with mosquito proteins critical for insect viability. The resulting human antimosquito antibodies are ingested in the mosquito's blood meal. The goal is to reduce parasite transmission by reducing the vitality or vector capability of the mosquito. An antimosquito vaccine that reduced the adult mosquito 's life span, so that parasite development would be interrupted, might have a significant effect on transmission.
Early studies reported that mortality of Anopheles stephensi was increased when these mosquitoes fed on rabbits that had been immunized with mos-
quito midgut extracts (Alger and Cabrera, 1972). Another mosquito, Aedes aegypti, when fed on rabbits immunized with whole Ae. aegypti extracts, suffered reduced reproductive capability (Sutherland and Ewen, 1974). Further work with Ae. aegypti fed on mice or rabbits immunized with selected mosquito body-part extracts demonstrated reduced viability of first generation offspring (Ramasamy et al., 1988) and increased mortality associated with the level and specificity of antibodies ingested (Hatfield, 1988a). Antibodies retained their immunological properties for two to three days and bound to the mosquito midgut epithelium (Hatfield, 1988b). Ramasamy and Ramasamy (1989) and Ramasamy et al. (1990) reported significant reductions in susceptibility to Ross River virus and Murray Valley encephalomyelitis virus in Ae. aegypti previously fed on rabbit blood-virus mixtures containing high levels of antibody against mosquito midgut extracts.
Recent work utilizing An. farauti (a vector of human malaria) and P. berghei (a rodent malaria parasite) showed that antibodies from mice immunized against mosquito midgut antigens appeared to prevent parasite ookinetes from penetrating the midgut of engorged mosquitoes (Ramasamy and Ramasamy, 1990). Interestingly, these same antibodies also reduced mosquito mortality rates, an undesirable outcome.
Although studies of antimosquito vaccines have used anthropophilic mosquitoes in unnatural animal host systems, they nevertheless hold promise for a new approach to reducing parasite transmission. This work is, however, in its early stages, and the opposing results obtained by Ramasamy and Ramasamy (1990) suggest that the immunological interactions among mosquitoes, host antibodies, and parasites are more complex than previously thought.
RESEARCH AGENDA
Identification of Mechanisms and Targets of Protective Immunity
Protective immunity can be induced in humans or experimental animals by repeated natural infection, by passive transfer of immune serum or cells, by immunization with radiation-attenuated parasites, or by immunization with subunit vaccines prepared by using purified parasite fractions, synthetic peptides or recombinant proteins. Identifying the mechanisms of such protective immunity will facilitate the development of subunit vaccines. Research on animal model systems will continue to be critical to the growth of basic knowledge in this area.
RESEARCH FOCUS: Continued use of animal model systems to identify and characterize mechanisms of protective antimalarial immunity.
RESEARCH FOCUS: Expanded studies in humans, including further immunization trials with radiation-attenuated sporozoites, to characterize the mechanisms of protective immunity.
Identification of antigens that are targets of protective immunity is incomplete. Successful approaches have included identification of antigens that are the target of protective immune responses; empiric screening of antigens, using monoclonal antibodies or recombinant DNA expression libraries; and identification of parasite molecules that perform an essential function and that are potentially accessible to immune attack. Further expansion of our knowledge of such target antigens is essential.
RESEARCH FOCUS: Continued identification of parasite antigens that are targets of protective immune responses.
Little is known about how malaria sporozoites invade liver cells. Our understanding of the process of merozoite invasion into erythrocytes is incomplete. The role of parasite proteases and other enzymes in parasite development is poorly characterized. The factors controlling transition from one parasite stage to the next are unknown. The parasite molecules involved in sequestration are poorly characterized. Tumor necrosis factor and other cytokines have been implicated in the pathogenesis of malaria disease, but little is known about the parasite molecules that induce such cytokines. Increased understanding of these and related aspects of parasite biology could provide important avenues for vaccine development.
RESEARCH FOCUS: Expanded studies of parasite biology.
Vaccine Formulation
The ideal malaria vaccine would be composed of multiple antigens from two or more stages of the parasite. The most useful of such proteins, the relevant epitopes in these molecules, the best way to combine them, and the appropriate adjuvants, dosage regimens, and routes of administration remain to be determined. It is unclear whether an effective immune response can be elicited against variant forms of the parasite by immunizing with conserved regions of these proteins.
RESEARCH FOCUS: Improved vaccine construction and delivery techniques, including the use of more potent adjuvants, time release preparations, live vectors, and better carrier proteins.
RESEARCH FOCUS: Better methods of assessing vaccine efficacy, with consideration given to conducting well-designed human vaccine trials at an early stage of antigen assessment.
In Vitro Assays
There is an immediate need for validated, reproducible, quantitative in vitro assays that correlate with and are predictive of protective immunity in humans. Such assays not only would permit candidate vaccines to be screened prior to human challenge studies, but also would facilitate field evaluations of vaccine effectiveness.
RESEARCH FOCUS: Development of in vitro tests predictive of protective immunity.
Culture Systems
Currently, only the asexual and sexual blood stages of P. falciparum can be easily cultured in vitro, and only in erythrocytes. The understanding of the basic biology and biochemistry of other species and stages of the malaria parasite could be dramatically improved with appropriate culture systems. P. vivax, for example, causes roughly half the world's malaria, but because it cannot be grown continuously in culture, relatively little research has gone into developing a vaccine against this parasite species. If sporozoites or blood-stage parasites could be cultured in the absence of contaminating cells, serious consideration could be given to developing whole-organism vaccines.
RESEARCH FOCUS: Methods for culturing P. falciparum sporozoites, improving culture techniques for P. falciparum liver-stage parasites, culturing the erythrocytic stages of P. falciparum in nonerythrocyte systems, and culturing P. vivax.
REFERENCES
Aggarwal, A., S. Kumar, R. Jaffe, D. Hone, M. Gross, and J. Sadoff. 1990. Oral Salmonella: malaria circumsporozoite recombinants induce specific CD8+ cytotoxic T cells. Journal of Experimental Medicine 172:1083-1090.
Aley, S. B., M. D. Bates, J. P. Tam, and M. R. Hollingdale. 1986. Synthetic peptides from the circumsporozoite proteins of Plasmodium falciparum and Plasmodium knowlesi recognize the human hepatoma cell line HepG2-A16 in vitro. Journal of Experimental Medicine 164:1915-1922.
Alger, N. E., and E. J. Cabrera. 1972. An increase in death rate of Anopheles stephensi fed on rabbits immunized with mosquito antigen. Journal of Economic Entomology 65:165-168.
Ballou, W. R., S. L. Hoffman, J. A. Sherwood, M. R. Hollingdale, F. A. Neva, W. T. Hockmeyer, D. M. Gordon, I. Schneider, R. A. Wirtz, J. F. Young, G. F. Wasserman, P. Reeve, C. L. Diggs, and J. D. Chulay. 1987. Safety and efficacy of a recombinant DNA Plasmodium falciparum sporozoite vaccine. Lancet 1:1277-1281.
Bate, C. A. W., J. Taverne, and J. H. L. Playfair. 1988. Malarial parasites induce tumour necrosis factor production by macrophages Immunology 64:227-231.
Bate, C. A. W., J. Taverne, and J. H. L. Playfair. 1989. Soluble malarial antigens are toxic and induce the production of tumour necrosis factor in vivo. Immunology 66:600-605.
Bate, C. A. W., J. Taverne, A. Dave, and J. H. L. Playfair. 1990. Malaria exoantigens induce T-independent antibody that blocks their ability to induce TNF. Immunology 70:315-320.
Beier, J. C., P. V. Perkins, F. Onyango, T. P. Gargan, C. N. Oster, R. E. Whitmire, D. K. Keoch, and C. R. Roberts. 1990. Characterization of malaria transmission by Anopheles (Diptera: Culicidae) in western Kenya in preparation for malaria vaccine trials. Journal of Medical Entomology 27:570-577.
Bouharoun-Tayoun, H., P. Attanath, A. Sabchareon, T. Chongsuphajaisiddhi, and P. Druihle. 1990. Antibodies that protect humans against Plasmodium falciparum blood stages do not on their own inhibit parasite growth and invasion in vitro, but act in cooperation with monocytes. Journal of Experimental Medicine 172:1633-1641.
Boyle, D. B., C. I. Newbold, C. C. Smith, and K. N. Brown. 1982. Monoclonal antibodies that protect in vivo against Plasmodium chabaudi recognize a 250,000-dalton parasite polypeptide. Infection and Immunity 38:94-102.
Brown, G.V. 1986. Prospects for a vaccine against malaria. Medical Journal of Australia 144:703-704.
Brown, K. N., W. Jarra, C. I. Newbold, and M. Schryer. 1985. Variability in parasite protein antigen structure and protective immunity to malaria. Annales de l'Institut Pasteur, Immunologie 136C:11-23.
Burns, J. M. Jr., W. R. Majarian, J. F. Young, T. M. Daly, and C. A. Long. 1989. A protective monoclonal antibody recognizes an epitope in the carboxyl-terminal cysteine-rich domain in the precursor of the major merozoite surface antigen of the rodent malarial parasite, Plasmodium yoelii. Journal of Immunology 143:2670-2676.
Cabantchik, Z. I., S. Kutner, M. Krugliak, and H. Ginsburg. 1983. Anion transport inhibitors as suppressors of Plasmodium falciparum growth in in vitro cultures. Molecular Pharmacology 23:92-99.
Camus, D., and T. J. Hadley. 1985. A Plasmodium falciparum antigen that binds to host erythrocytes and merozoites. Science 230:553-556.
Carter, R., and D. H. Chen. 1976. Malaria transmission blocked by immunisation with gametes of the malaria parasite. Nature 263:57-60.
Carter, R., N. Kumar, I. Quakyi, M. Good, K. Mendis, P. Graves, and L. Miller. 1988. Immunity to sexual stages of malaria parasites. Progress in Allergy 41:193-214.
Carter, R., P. M. Graves, I. A. Quakyi, and M. F. Good. 1989. Restricted or absent immune responses in human populations to Plasmodium falciparum gamete antigens that are targets of malaria transmission-blocking antibodies. Journal of Experimental Medicine 169:135-147.
Charoenvit, Y., M. F. Leef, L. F. Yuan, M. Sedegah, and R. L. Beaudoin. 1987. Characterization of Plasmodium yoelii monoclonal antibodies directed against stage-specific sporozoite antigens. Infection and Immunity 55:604-608.
Charoenvit, Y., W. E. Collins, T. R. Jones, P. Millet, L. Yuan, G. H. Campbell, R. L. Beaudoin, J. R. Broderson, and S. L. Hoffman. 1991a. Inability of malaria vaccine to induce antibodies to a protective epitope within its sequence. Science 251:668-671.
Charoenvit, Y., S. Mellouk, C. Cole, R. Bechara, M. F. Leef, M. Sedegah, L. F. Yuan, F. A. Robey, R. L. Beaudoin, and S. L. Hoffman. 1991b. Monoclonal, but not polyclonal, antibodies, protect against Plasmodium yoelii sporozoites. Journal of Immunology 146:1020-1025.
Chen, D. H., R. E. Tigelaar, and F. I. Weinbaum. 1977. Immunity to sporozoite-induced malaria infection in mice. I. The effect of immunization of T and B cell-deficient mice. Journal of Immunology 118:1322-1327.
Cheung, A., A. R. Shaw, J. Leban, and L. H. Perrin. 1985. Cloning and expression in Escherichia coli of a surface antigen of Plasmodium falciparum merozoites. EMBO Journal 4:1007-1012.
Cheung, A., J. Leban, A. R. Shaw, B. Merkli, J. Stocker, C. Chizzolini, C. Sander, and L. H. Perrin. 1986. Immunization with synthetic peptides of a Plasmodium falciparum surface antigen induces antimerozoite antibodies. Proceedings of the National Academy of Sciences of the United States of America 83:8328-8332.
Chulay, J. D. 1989. Development of sporozoite vaccines for malaria. Transactions of the Royal Society of Tropical Medicine and Hygiene 83(Suppl.):61-66.
Chulay, J. D., and C. F. Ockenhouse. 1990. Host receptors for malaria-infected erythrocytes. American Journal of Tropical Medicine and Hygiene 43(Suppl):6-14.
Chulay, J. D., M. Aikawa, C. Diggs, and J. D. Haynes. 1981. Inhibitory effects of immune monkey serum on synchronized Plasmodium falciparum cultures. American Journal of Tropical Medicine and Hygiene 30:12-19.
Chulay, J. D., J. A. Lyon, J. D. Haynes, A. I. Meierovics, C. T. Atkinson, and M. Aikawa. 1987. Monoclonal antibody characterization of Plasmodium falciparum antigens in immune complexes formed when schizonts rupture in the presence of immune serum. Journal of Immunology 139:2768-2774.
Clyde, D. F., V. C. McCarthy, R. M. Miller, and R. B. Hornick. 1973a. Specificity of protection of man immunized against sporozoite-induced falciparum malaria. American Journal of the Medical Sciences 266:398-403.
Clyde, D. F., H. Most, V. C. McCarthy, and J. P. Vanderberg. 1973b. Immunization of man against sporozoite-induced falciparum malaria American Journal of the Medical Sciences 266:169-177.
Clyde, D. F., V. C. McCarthy, R. M. Miller, and W. E. Woodward. 1975. Immunization of man against falciparum and vivax malaria by use of attenuated sporozoites. American Journal of Tropical Medicine and Hygiene 24:397-401.
Cohen, S., I. A. McGregor, and S. P. Carrington. 1961. Gamma globulin and acquired immunity to human malaria. Nature 192:733-737.
Collins, W. E., R. S. Nussenzweig, W. R. Ballou, T. K. I. I. Ruebush, E. H. Nardin, J. D. Chulay, W. R. Majarian, J. F. Young, G. F. Wasserman, I. Bathurst, H. L. Gibson, P. J. Barr, S. L. Hoffman, S. S. Wasserman, J. R. Broderson, J. C. Skinner, P. M. Procell, V. K. Filipski, and C. L. Wilson. 1989. Immunization of Saimiri sciureus boliviensis with recombinant vaccines based on the circumsporozoite protein of Plasmodium vivax. American Journal of Tropical Medicine and Hygiene 40:455-464.
Dame, J. B., J. L. Williams, T. F. McCutchan, J. L. Weber, R. A. Wirtz, W. T. Hockmeyer, W. L. Maloy, J. D. Haynes, I. Schneider, D. Roberts, G. S. Sanders, E. P. Reddy, C. L. Diggs, and L. H. Miller. 1984. Structure of the gene encoding the immunodominant surface antigen on the sporozoite of the human malaria parasite Plasmodium falciparum. Science 225:593-599.
David, P. H., M. Hommel, L. H. Miller, I. J. Udeinya, and L. D. Oligino. 1983. Parasite sequestration in Plasmodium falciparum malaria: spleen and antibody modulation of cytoadherence of infected erythrocytes. Proceedings of the National Academy of Sciences of the United States of America 80:5075-5079.
de Groot, A. S., A. H. Johnson, W. L. Maloy, I. A. Quakyi, E. M. Riley, A. Menon, S. M. Banks, J. A. Berzofsky, and M. F. Good. 1989. Human T cell recognition of polymorphic epitopes from malaria circumsporozoite protein. Journal of Immunology 142:4000-4005.
Del Giudice, G., J. A. Cooper, J. Merino, A. S. Verdini, A. Pessi, A. R. Togna, H. D. Engers, G. Corradin, and P.-H. Lambert. 1986. The antibody response in mice to carrier-free synthetic polymers of Plasmodium falciparum circumsporozoite repetitive epitope is I-Ab-restricted: possible implications for malaria vaccines. Journal of Immunology 137:2952-2955.
Del Giudice, G., D. Grillot, L. Renia, I. Mullere, G. Corradin, J. A. Louis, D. Mazier, and P.-H. Lambert. 1990. Peptide-primed CD4+ cells and malaria sporozoites. Immunology Letters 25:59-64.
Deres, K., H. Schild, K.-H. Wiesmuller, G. Jung, and H.-G. Rammensee. 1989. In vivo priming of virus-specific cytotoxic T lymphocytes with synthetic lipopeptide vaccine. Nature 342:561-564.
Egan, J. E., J. L. Weber, W. R. Ballou, M. R. Hollingdale, W. R. Majarian, D. M. Gordon, W. L. Maloy, S. L. Hoffman, R. A. Wirtz, I. Schneider, G. R. Woollett, J. F. Young, and W. T. Hockmeyer. 1987. Efficacy of murine malaria sporozoite vaccines: implications for human vaccine development. Science 236:453-456.
Elford, B. C., J. D. Haynes, J. D. Chulay, and R. J. M. Wilson. 1985. Selective stage-specific changes in the permeability to small hydrophilic solutes of human erythrocytes infected with Plasmodium falciparum. Molecular and Biochemical Parasitology 16:43-60.
Etlinger, H. M., D. Gillessen, H.-W. Lahm, H. Matile, H.-J. Schonfeld, and A. Trzeciak. 1990. Use of prior vaccinations for the development of new vaccines. Science 249:423-425.
Ferreira, A., L. Schofield, V. Enea, H. Shellekens, P. Van der Meide, W. E. Collins, R. S. Nussenzweig, and V. Nussenzweig. 1986. Inhibition of development of exoerythrocytic forms of malaria parasites by gamma-interferon
. Science 232:881-884.
Galinski, M. R., D. E. Arnot, A. H. Cochrane, J. W. Barnwell, R. S. Nussenzweig, and V. Enea. 1987. The circumsporozoite gene of the Plasmodium cynomolgi complex. Cell 48:311-319.
Good, M. F. 1988. T cells, T sites, and malaria immunity—further optimism for vaccine development. Journal of Immunology 140:1715-1716.
Good, M. F., J. A. Berzofsky, W. L. Maloy, Y. Hayashi, N. Fujii, W. T. Hockmeyer, and L. H. Miller. 1986. Genetic control of the immune response in mice to a Plasmodium falciparum sporozoite vaccine. Widespread nonresponsiveness to single malaria T epitope in highly repetitive vaccine. Journal of Experimental Medicine 164:655-660.
Good, M. F., W. L. Maloy, M. N. Lunde, H. Margalit, J. L. Cornette, G. L. Smith, B. Moss, L. H. Miller, and J. A. Berzofsky. 1987. Construction of synthetic immunogen: use of new T-helper epitope on malaria circumsporozoite protein. Science 235:1059-1062.
Good, M. F., J. A. Berzofsky, and L. H. Miller. 1988a. The T cell response to the malaria circumsporozoite protein: an immunological approach to vaccine development. Pp. 663-688 in Annual Review of Immunology, Paul, W. E., C. G. Fathman, R. Germain, and H. Metzger, eds. Palo Alto: Annual Reviews, Inc.
Good, M. F., L. H. Miller, S. Kumar, I. A. Quakyi, D. Keister, J. H. Adams, B. Moss, J. A. Berzofsky, and R. Carter. 1988b. Limited immunological recognition of critical malaria vaccine candidate antigens. Science 242:574-577.
Good, M. F., D. Pombo, W. L. Maloy, V. F. de la Cruz, L. H. Miller, and J. A. Berzofsky. 1988c. Parasite polymorphism present within minimal T cell epitopes of Plasmodium falciparum circumsporozoite protein. Journal of Immunology 140:1645-1650.
Good, M. F., D. Pombo, I. A. Quakyi, E. M. Riley, R. A. Houghten, A. Menon, D. W. Alling, J. A. Berzofsky, and L. H. Miller. 1988d. Human T-cell recognition of the circumsporozoite protein of Plasmodium falciparum: immunodominant T-cell domains map to the polymorphic regions of the molecule. Proceedings of the National Academy of Sciences of the United States of America 85:1199-1203.
Gordon, D. M., T. M. Cosgriff, I. Schneider, G. F. Wasserman, W. R. Majarian, M. R. Hollingdale, and J. D. Chulay. 1990. Safety and immunogenicity of a Plasmodium vivax sporozoite vaccine. American Journal of Tropical Medicine and Hygiene 42:527-531.
Graves, P. M., R. Carter, T. R. Burkot, J. Rener, D. C. Kaushal, and J. L. Williams. 1985. Effects of transmission-blocking monoclonal antibodies on different isolates of Plasmodium falciparum. Infection and Immunity 48:611-616.
Graves, P. M., R. Carter, T. R. Burkot, I. A. Quakyi, and N. Kumar. 1988. Antibodies to Plasmodium falciparum gamete surface antigens in Papua New Guinea sera. Parasite Immunology 10:209-218.
Guerin-Marchand, C., P. Druilhe, B. Galey, A. Londono, J. Patarpotikul, R. L. Beaudoin, C. Dubeaux, A. Tartar, O. Mercereau-Puijalon, and G. Langsley. 1987. A liver-stage-specific antigen of Plasmodium falciparum characterized by gene cloning. Nature 329:164-167.
Gwadz, R.W. 1976. Malaria: successful immunization against the sexual stages of Plasmodium gallinaceum. Science 193:1150-1151.
Gwadz, R. W., A. H. Cochrane, V. Nussenzweig, and R. S. Nussenzweig. 1979. Preliminary studies on vaccination of rhesus monkeys with irradiated sporozoites of Plasmodium knowlesi and characterisation of surface antigens of these parasites. Bulletin of the World Health Organization 57(Suppl 1):165-173.
Hadley, T. J., and L. H. Miller. 1988. Invasion of erythrocytes by malaria parasites: erythrocyte ligands and parasite receptors. Progress in Allergy 41:49-71.
Haldar, K., M. A. J. Ferguson, and G. A. M. Cross. 1985. Acylation of a Plasmodium falciparum merozoite surface antigen via sn-1,2-diacyl glycerol. Journal of Biological Chemistry 260:4969-4974.
Hall, R., J. E. Hyde, M. Goman, D. L. Simmons, I. A. Hope, M. Mackay, J. Scaife, B. Merkli, R. Richle, and J. Stocker. 1984. Major surface antigen gene of a human malaria parasite cloned and expressed in bacteria. Nature 311:379-382.
Harte, P. G., N. C. Rogers, and G. A. T. Targett. 1985. Role of T cells in preventing transmission of rodent malaria. Immunology 56:1-7.
Hatfield, P. R. 1988a. Anti-mosquito antibodies and their effects on feeding, fecundity and mortality of Aedes aegypti. Medical and Veterinary Entomology 2:331-338.
Hatfield, P. R. 1988b. Detection and localization of antibody ingested with a mosquito bloodmeal Medical and Veterinary Entomology 2:339-345.
Hedstrom, R. C., J. R. Campbell, M. L. Leef, Y. Charoenvit, M. Carter, M. Sedegah, R. L. Beaudoin, and S. L. Hoffman. 1990. A malaria sporozoite surface antigen distinct from the circumsporozoite protein. Bulletin of the World Health Organization 68(Suppl):152-157.
Herrington, D. A., D. F. Clyde, G. Losonsky, M. Cortesia, J. R. Murphy, J. Davis, S. Baqar, A. M. Felix, E. P. Heimer, D. Gillessen, E. Nardine, R. S. Nussenzweig, V. Nussenzweig, M. R. Hollingdale, and M. M. Levine. 1987. Safety and immunogenicity in man of a synthetic peptide malaria vaccine against Plasmodium falciparum sporozoites. Nature 328:257-259.
Hoffman, S. L., C. N. Oster, C. V. Plowe, G. R. Woollett, J. C. Beier, J. D. Chulay, R. A. Wirtz, M. R. Hollingdale, and M. Mugambi. 1987. Naturally acquired antibodies to sporozoites do not prevent malaria: vaccine development implications. Science 237:639-642.
Hoffman, S. L., J. A. Berzofsky, D. Isenbarger, E. Zeltser, W. R. Majarian, M. Gross, and W. R. Ballou. 1989a. Immune response gene regulation of immunity to Plasmodium berghei sporozoites and circumsporozoite protein vaccines: overcoming genetic restriction with whole organism and subunit vaccines. Journal of Immunology 142:3581-3584.
Hoffman, S. L., D. Isenbarger, G. W. Long, M. Sedegah, A. Szarfman, L. Waters, M. R. Hollingdale, P. H. van der Meide, D. S. Finbloom, and W. R. Ballou. 1989b. Sporozoite vaccine induces genetically restricted T cell elimination of malaria from hepatocytes. Science 244:1078-1081.
Hoffman, S. L., C. N. Oster, C. Mason, J. C. Beier, J. A. Sherwood, W. R. Ballou, M. Mugambi, and J. D. Chulay. 1989c. Human lymphocyte proliferative response to a sporozoite T cell epitope correlates with resistance to falciparum malaria. Journal of Immunology 142:1299-1303.
Holder, A.A. 1988. The precursor to major merozoite surface antigens: structure and role in immunity. Progress in Allergy 41:72-97.
Holder, A. A., and R. R. Freeman. 1981. Immunization against blood-stage rodent malaria using purified parasite antigens. Nature 294:361-364.
Holder, A. A., and R. R. Freeman. 1984. Protective antigens of rodent and human bloodstage malaria. Philosophical Transactions of the Royal Society of London, Series B: Biological Sciences 307:171-177.
Holder, A. A., M. J. Lockyer, K. G. Odink, J. S. Sandhu, V. Riveros-Moreno, S. C. Nicholls, Y. Hillman, L. S. Davey, M. L. Tizard, R. T. Schwarz, and R. R. Freeman. 1985. Primary structure of the precursor to the three major surface antigens of Plasmodium falciparum merozoites. Nature 317:270-273.
Hollingdale, M. R., E. H. Nardin, S. Tharavanij, A. L. Schwartz, and R. S. Nussenzweig. 1984. Inhibition of entry of Plasmodium falciparum and P. vivax sporozoites into cultured cells; an in vitro assay of protective antibodies. Journal of Immunology 132:909-913.
Hollingdale, M. R., M. Aikawa, C. T. Atkinson, W. R. Ballou, G. Chen, J. Li, J. F. G. M. Meis, B. Sina, C. Wright, and J. Zhu. 1990. Non-CS pre-erythrocytic protective antigens. Immunology Letters 25:71-76.
Howard, R. J., and A. D. Gilladoga. 1989. Molecular studies related to the pathogenesis of cerebral malaria Blood 74:2603-2618.
Howard, R. J., S. Uni, M. Aikawa, S. B. Aley, J. H. Leech, A. M. Lew, T. E. Wellems, J. Rener, and D. W. Taylor. 1986. Secretion of a malarial histidinerich protein (PfHRP II) from Plasmodium falciparum-infected erythrocytes. Journal of Cell Biology 103:1269-1277.
Howard, R. J., S. M. Handunnetti, T. Hasler, A. Gilladoga, J. C. de Aguiar, B. L. Pasloske, K. Morehead, G. R. Albrecht, and M. R. van Schravendijk. 1990. Surface molecules on Plasmodium falciparum-infected erythrocytes involved in adherence. American Journal of Tropical Medicine and Hygiene 43(Suppl.):15-29.
Jahiel, R. I., R. S. Nussenzweig, J. Vanderberg, and J. Vilcek. 1968a. Anti-malarial effect of interferon inducers at different stages of development of Plasmodium berghei in the mouse. Nature 220:710-711.
Jahiel, R. I., J. Vilcek, R. Nussenzweig, and J. Vanderberg. 1968b. Interferon inducers protect mice against Plasmodium berghei malaria. Science 161:802-804.
Jungery, M. 1985. Studies on the biochemical basis of the interaction of the merozoites of Plasmodium falciparum and the human red cell. Transactions of the Royal Society of Tropical Medicine and Hygiene 79:591-597.
Kaslow, D. C. 1990. Immunogenicity of Plasmodium falciparum sexual stage antigens: implications for the design of a transmission blocking vaccine. Immunology Letters 25:83-86.
Kaslow, D. C., I. A. Quakyi, C. Syin, M. G. Raum, D. B. Keister, J. E. Coligan, T. F. McCutchan, and L. H. Miller. 1988. A vaccine candidate from the sexual stage of human malaria that contains EGF-like domains. Nature 333:74-76.
Kaslow, D. C., I. A. Quakyi, and D. B. Keister. 1989. Minimal variation in a vaccine candidate from the sexual stage of Plasmodium falciparum. Molecular and Biochemical Parasitology 32:101-103.
Kaslow, D. C., S. N. Isaacs, I. A. Quakyi, R. W. Gwadz, B. Moss, and D. B. Keister
. 1991. Induction of Plasmodium falciparum transmission-blocking antibodies by recombinant vaccinia virus. Science 252:1310-1313.
Khusmith, S., and P. Druihle. 1983. Antibody-dependent ingestion of P. falciparum merozoites by human blood monocytes. Parasite Immunology 5:357-368.
Khusmith, S., Y. Charoenvit, S. Kumar, M. Sedegah, R. L. Beaudoin, and S. L. Hoffman. 1991. Protection against malaria by vaccination with sporozoite surface protein 2 plus CS protein. Science 252:715-718.
Kumar, S., L. H. Miller, I. A. Quakyi, D. B. Keister, R. A. Houghten, W. L. Maloy, B. Moss, J. A. Berzofsky, and M. F. Good. 1988. Cytotoxic T cells specific for the circumsporozoite protein of Plasmodium falciparum. Nature 334:258-260.
Leech, J. H., J. W. Barnwell, L. H. Miller, and R. J. Howard. 1984. Identification of a strain-specific malarial antigen exposed on the surface of Plasmodium falciparum-infected erythrocytes. Journal of Experimental Medicine 159:1567-1575.
Lew, A. M., C. J. Langford, R. F. Anders, D. J. Kemp, A. Saul, C. Fardoulys, M. Geysen, and M. Sheppard. 1989. A protective monoclonal antibody recognizes a linear epitope in the precursor to the major merozoite antigens of Plasmodium chabaudi adami. Proceedings of the National Academy of Sciences of the United States of America 86:3768-3772.
Lockyer, M. J., and R. T. Schwarz. 1987. Strain variation in the circumsporozoite protein gene of Plasmodium falciparum. Molecular and Biochemical Parasitology 22:101-108.
Lussow, A. R., G. Del Giudice, L. Renia, D. Mazier, J. P. Verhave, A. S. Verdini, A. Pessi, J. A. Louis, and P. H. Lambert. 1990. Use of a tuberculin purified protein derivative—Asn-Ala-Asn-Pro conjugate—in bacillus Calmette-Guerin primed mice overcomes H-2 restriction of the antibody response and avoids the need for adjuvants. Proceedings of the National Academy of Sciences of the United States of America 87:2960-2964.
Lyon, J. A., A. W. Thomas, T. Hall, and J. D. Chulay. 1989. Specificities of antibodies that inhibit merozoite dispersal from malaria-infected erythrocytes. Molecular and Biochemical Parasitology 36:77-86.
Mackay, M., M. Goman, N. Bone, J. E. Hyde, J. Scaife, U. Certa, H. Stunnenberg, and H. Bujard. 1985. Polymorphism of the precursor for the major surface antigens of Plasmodium falciparum merozoites: studies at the genetic level. EMBO Journal 4:3823-3829.
Maheshwari, R. K., C. W. Czarniecki, G. P. Dutta, S. K. Puri, B. N. Dhawan, and R. M. Friedman. 1986. Recombinant human gamma interferon inhibits simian malaria. Infection and Immunity 53:628-630.
Majarian, W. R., T. M. Daly, W. P. Weidanz, and C. A. Long. 1984. Passive immunization against murine malaria with an IgG3 monoclonal antibody. Journal of Immunology 132:3131-3137.
Malik, A., J. E. Egan, R. Houghten, and S. L. Hoffman. 1991. Human cytotoxic T lymphocytes against the Plasmodium falciparum circumsporozoite protein. Proceedings of the National Academy of Sciences of the United States of America 88:3300-3304.
Marsh, K., and R. J. Howard. 1986. Antigens induced on erythrocytes by P. falciparum: expression of diverse and conserved determinants. Science 231:150.
Mazier, D., S. Mellouk, R. L. Beaudoin, B. Texier, P. Druilhe, W. Hockmeyer, J. Trosper,
C. Paul, Y. Charoenvit, J. Young, F. Miltgen, L. Chedid, J. P. Chigot, B. Galley, O. Brandicourt, and M. Gentilini. 1986. Effect of antibodies to recombinant and synthetic peptides on P. falciparum sporozoites in vitro. Science 231:156-159.
McBride, J. S., C. I. Newbold, and R. Anand. 1985. Polymorphism of a high molecular weight schizont antigen of the human malaria parasite Plasmodium falciparum. Journal of Experimental Medicine 161:160-180.
McGregor, I. A., S. P. Carrington, and S. Cohen. 1963. Treatment of East African P. falciparum malaria with West African human gammaglobulin. Transactions of the Royal Society of Tropical Medicine and Hygiene 57:170-175.
Mellouk, S., R. K. Maheshwari, A. Rhodes-Feuillette, R. L. Beaudoin, N. Berbiguier, H. Matile, F. Miltgen, I. Landau, S. Pied, J. P. Chigot, R. M. Friedman, and D. Mazier. 1987. Inhibitory activity of interferons and interleukin 1 on the development of Plasmodium falciparum in human hepatocyte cultures. Journal of Immunology 139:4192-4195.
Mendis, K. N., Y. D. Munesinghe, Y. N. Y. deSilva, I. Keragalla, and R. Carter. 1987. Malaria transmission-blocking immunity induced by natural infections of Plasmodium vivax in humans. Infection and Immunity 55:369-372.
Miller, L. H., M. Aikawa, and J. A. Dvorak. 1975. Malaria (Plasmodium knowlesi) merozoites: immunity and the surface coat. Journal of Immunology 114:1237-1242.
Miller, L. H., S. J. Mason, D. F. Clyde, and M. H. McGinniss. 1976. The resistance factor to Plasmodium vivax in blacks. The Duffy-blood-group genotype, FyFy. New England Journal of Medicine 295:302-304.
Mitchell, G. H., T. J. Hadley, M. H. McGinniss, F. W. Klotz, and L. H. Miller. 1986. Invasion of erythrocytes by Plasmodium falciparum malaria parasites: evidence for receptor heterogeneity and two receptors Blood 67:1519-1521.
Murphy, V. F., W. C. Rowan, M. J. Page, and A. A. Holder. 1990. Expression of hybrid malaria antigens in insect cells and their engineering for correct folding and secretion. Parasitology 100(Pt. 2):177-183.
Nussenzweig, R. S., J. Vanderberg, H. Most, and C. Orton. 1967. Protective immunity induced by the injection of X-irradiated sporozoites of Plasmodium berghei. Nature 216:160-162.
Ockenhouse, C. F., S. Schulman, and H. L. Shear. 1984. Induction of crisis forms in the human malaria parasite Plasmodium falciparum by gamma-interferon-activated, monocyte-derived macrophages. Journal of Immunology 133:1601-1608.
Orlandi, P. A., B. K. L. Sim, J. D. Chulay, and J. D. Haynes. 1990. Characterization of the 175-kilodalton erythrocyte binding antigen of Plasmodium falciparum. Molecular and Biochemical Parasitology 40:285-294.
Patarroyo, M. E., P. Romero, M. L. Torres, P. Clavijo, A. Moreno, A. Martinez, R. Rodriguez, F. Guzman, and E. Cabezas. 1987. Induction of protective immunity against experimental infection with malaria using synthetic peptides. Nature 328:629-632.
Patarroyo, M. E., R. Amador, P. Clavijo, A. Moreno, F. Guzman, P. Romero, R. Tascon, A. Franco, L. A. Murillo, G. Ponton, and G. Trujillo. 1988. A synthetic vaccine protects humans against challenge with asexual blood stages of Plasmodium falciparum malaria. Nature 332:158-161.
Peiris, J. S. M., S. Premawansa, M. B. R. Ranawaka, P. V. Udagama, Y. D. Munasinghe, M. V. Nanayakkara, C. P. Gamage, R. Carter, P. H. David, and K. N. Mendis. 1988. Monoclonal and polyclonal antibodies both block and enhance transmission of Plasmodium vivax malaria. American Journal of Tropical Medicine and Hygiene 39:26-32.
Perkins, M. E. 1989. Erythrocyte invasion by the malarial merozoite: recent advances. Experimental Parasitology 69:94-99.
Perrin, L. H., E. Ramirez, P. H. Lambert, and P. A. Miescher. 1981. Inhibition of P. falciparum growth in human erythrocytes by monoclonal antibodies. Nature 289:301-303.
Peterson, M. G., R. L. Coppel, P. McIntyre, C. J. Langford, G. Woodrow, G. V. Brown, R. F. Anders, and D. J. Kemp. 1988a. Variation in the precursor to the major merozoite surface antigens of Plasmodium falciparum. Molecular and Bio-chemical Parasitology 27:291-302.
Peterson, M. G., R. L. Coppel, M. B. Moloney, and D. J. Kemp. 1988b. Third form of the precursor to the major merozoite surface antigens of Plasmodium falciparum. Molecular and Cellular Biology 8:2664-2667.
Playfair, J. H. L., J. Taverne, C. A. W. Bate, and J. B. de Souza. 1990. The malaria vaccine: antiparasite or anti-disease? Immunology Today 11:25-27.
Potocnjak, P., N. Yoshida, R. S. Nussenzweig, and V. Nussenzweig. 1980. Monovalent fragments (Fab) of monoclonal antibodies to a sporozoite surface antigen (Pb44) protect mice against malarial infection. Journal of Experimental Medicine 151:1504-1513.
Quakyi, I. A., R. Carter, J. Rener, N. Kumar, M. F. Good, and L. H. Miller. 1987. The 230-kDa gamete surface protein of Plasmodium falciparum is also a target of transmission-blocking antibodies. Journal of Immunology 139:4213-4217.
Quakyi, I. A., L. N. Otoo, D. Pombo, L. Y. Sugars, A. Menon, A. S. DeGroot, A. Johnson, D. Alling, L. H. Miller, and M. F. Good. 1989. Differential non-responsiveness in humans of candidate Plasmodium falciparum vaccine antigens. American Journal of Tropical Medicine and Hygiene 41:125-134.
Ramasamy, M. S., and R. Ramasamy. 1989. Effect of host anti-mosquito antibodies on mosquito physiology and mosquito-pathogen interactions. Pp. 142-148 in Host Regulated Developmental Mechanisms in Vector Arthropods, Borovsky, D., and A. Spielman, eds. University of Florida-IFAS: Vero Beach.
Ramasamy, M. S., and R. Ramasamy. 1990. Effect of anti-mosquito antibodies on the infectivity of the rodent malaria parasite Plasmodium berghei to Anophelesfarauti. Medical and Veterinary Entomology 4:161-166.
Ramasamy, M. S., R. Ramasamy, B. H. Kay, and C. Kidson. 1988. Anti-mosquito antibodies decrease reproductive capacity of Aedes aegypti. Medical and Veterinary Entomology 2:87-93.
Ramasamy, M. S., M. Sands, B. H. Kay, I. D. Fanning, G. W. Lawrence, and R. Ramasamy. 1990. Anti-mosquito antibodies reduce the susceptibility of Aedesaegypti to arbovirus infection. Medical and Veterinary Entomology 4:49-55.
Rener, J., P. M. Graves, R. Carter, J. L. Williams, and T. R. Burkot. 1983. Target antigens of transmission blocking immunity on gametes of Plasmodium falciparum. Journal of Experimental Medicine 158:976-981.
Rickman, L. S., D. M. Gordon, R. Wistar, Jr., U. Krzych, M. Gross, M. R. Hollingdale,
J. E. Egan, J. D. Chulay, and S. L. Hoffman. 1991. Use of adjuvant containing mycobacterial cell-wall skeleton, monophosphoryl lipid A, and squalene in malaria circumsporozoite protein vaccine Lancet 337:998-1001.
Rieckmann, K. H., P. E. Carson, R. L. Beaudoin, J. S. Cassells, and K. W. Sell. 1974. Sporozoite induced immunity in man against an Ethiopian strain of Plasmodium falciparum. Transactions of the Royal Society of Tropical Medicine and Hygiene 68:258-259.
Rieckmann, K. H., R. L. Beaudoin, J. S. Cassells, and K. W. Sell. 1979. Use of attenuated sporozoites in the immunization of human volunteers against falciparum malaria. Bulletin of the World Health Organization 57(Suppl 1):261-265.
Romero, P., J. L. Maryanski, G. Corradin, R. S. Nussenzweig, V. Nussenzweig, and F. Zavala. 1989. Cloned cytotoxic T cells recognize an epitope in the circumsporozoite protein and protect against malaria. Nature 341:323-326.
Rosenberg, R., R. A. Wirtz, D. E. Lanar, J. Sattabongkot, T. Hall, A. P. Waters, and C. Prasittisuk. 1989. Circumsporozoite protein heterogeneity in the human malaria parasite Plasmodium vivax. Science 245:973-976.
Sadoff, J. C., W. R. Ballou, L. S. Baron, W. R. Majarian, R. N. Brey, W. T. Hockmeyer, J. F. Young, S. J. Cryz, J. Ou, G. H. Lowell, and J. D. Chulay. 1988. Oral Salmonella typhimurium vaccine expressing circumsporozoite protein protects against malaria Science 240:336-338.
Saul, A., P. Myler, L. Schofield, and C. Kidson. 1984. A high molecular weight antigen in Plasmodium falciparum recognized by inhibitory monoclonal antibodies. Parasite Immunology 6:39-50.
Saul, A., J. Cooper, L. Ingram, R. F. Anders, and G. V. Brown. 1985. Invasion of erythrocytes in vitro by Plasmodium falciparum can be inhibited by monoclonal antibody directed against an S antigen Parasite Immunology 7:587-593.
Schmidt-Ullrich, R., J. Lightholder, and M. T. Monroe. 1983. Protective Plasmodium knowlesi Mr 74,000 antigen in membranes of schizont-infected rhesus erythrocytes Journal of Experimental Medicine 158:146-158.
Schmidt-Ullrich, R., J. Brown, H. Whittle, and P.-S. Lin. 1986. Human-human hybridomas secreting monoclonal antibodies to the Mr 195,000 Plasmodium falciparum blood stage antigen. Journal of Experimental Medicine 163:179-188.
Schofield, L., G. R. Bushell, J. A. Cooper, A. J. Saul, J. A. Upcroft, and C. Kidson. 1986. A rhoptry antigen of Plasmodium falciparum contains conserved and variable epitopes recognized by inhibitory monoclonal antibodies. Molecular and Biochemical Parasitology 18:183-195.
Schofield, L., A. Ferreira, R. Altszuler, V. Nussenzweig, and R. S. Nussenzweig. 1987a. Interferon-gamma inhibits the intrahepatocytic development of malaria parasites in vitro. Journal of Immunology 139:2020-2025.
Schofield, L., J. Villaquiran, A. Ferreira, H. Schellekens, R. Nussenzweig, and V. Nussenzweig. 1987b. Gamma interferon, CD8+ T cells and antibodies required for immunity to malaria sporozoites. Nature 330:664-666.
Sharma, S., P. Svec, G. H. Mitchell, and G. N. Godson. 1985. Diversity of circumsporozoite antigen genes from two strains of the malarial parasite Plasmodium knowlesi. Science 229:779-782.
Siddiqui, W. A., L. Q. Tam, K. J. Kramer, G. S. N. Hui, S. E. Case, K. M. Yamaga,
S. P. Chang, E. B. T. Chan, and S.-C. Kan. 1987. Merozoite surface coat precursor protein completely protects Aotus monkeys against Plasmodium falciparum malaria. Proceedings of the National Academy of Sciences of the United States of America 84:3014-3018.
Sieber, K.-P., M. Huber, D. Kaslow, S. M. Banks, M. Torii, M. Aikawa, and L. H. Miller. 1991. The peritrophic membrane as a barrier: its penetration by Plasmodium gallinaceum and the effect of a monoclonal antibody to ookinetes. Experimental Parasitology 72:145-156.
Sim, B. K. L., P. A. Orlandi, J. D. Haynes, F. W. Klotz, J. M. Carter, D. Camus, M. E. Zegans, and J. D. Chulay. 1990. Primary structure of the 175K Plasmodium falciparum erythrocyte binding antigen and identification of a peptide which elicits antibodies that inhibit malaria merozoite invasion. Journal of Cell Biology 111:1877-1884.
Sutherland, G. B., and A. B. Ewen. 1974. Fecundity decrease in mosquitoes ingesting blood from specifically sensitized mammals. Journal of Insect Physiology 20:655-660.
Takahashi, H., T. Takeshita, B. Morein, S. Putney, R. N. Germain, and J. A. Berzofsky. 1990. Induction of CD8+ cytotoxic T cells by immunization with purified HIV-1 envelope protein in ISCOMs. Nature 344:873-875.
Tam, J. P., P. Clavijo, Y. A. Lu, V. Nussenzweig, R. Nussenzweig, and F. Zavala. 1990. Incorporation of T and B epitopes of the circumsporozoite protein in a chemically defined synthetic vaccine against malaria. Journal of Experimental Medicine 171:299-306.
Tanabe, K., M. Mackay, M. Goman, and J. G. Scaife. 1987. Allelic dimorphism in a surface antigen gene of the malaria parasite Plasmodium falciparum. Journal of Molecular Biology 195:273-287.
Targett, G. A. T., P. G. Harte, S. Eida, N. C. Rogers, and C. S. L. Ong. 1990. Plasmodium falciparum sexual stage antigens: immunogenicity and cell-mediated responses Immunology Letters 25:77-82.
Taverne, J., C. A. Bate, D. Kwiatkowski, P. H. Jakobsen, and J. H. Playfair. 1990. Two soluble antigens of Plasmodium falciparum induce tumor necrosis factor release from macrophages. Infection and Immunity 58:2923-2928.
Thomas, A. W., J. A. Deans, G. H. Mitchell, T. Alderson, and S. Cohen. 1984. The Fab fragments of monoclonal IgG to a merozoite surface antigen inhibit Plasmodium knowlesi invasion of erythrocytes. Molecular and Biochemical Parasitology 13:187-199.
Tsuji, M., P. Romero, R. S. Nussenzweig, and F. Zavala. 1990. CD4+ cytolytic T cell clone confers protection against murine malaria Journal of Experimental Medicine 172:1353-1357.
Udeinya, I. J., L. H. Miller, I. A. McGregor, and J. B. Jensen. 1983. Plasmodium falciparum strain-specific antibody blocks binding of infected erythrocytes to amelanotic melanoma cells. Nature 303:429-431.
Udomsangpetch, R., M. Aikawa, K. Berzins, M. Wahlgren, and P. Perlmann. 1989. Cytoadherence of knobless Plasmodium falciparum-infected erythrocytes and its inhibition by a human monoclonal antibody Nature 338:763-765.
Vermeulen, A. N., T. Ponnudurai, P. J. A. Beckers, J.-P. Verhave, M. A. Smits, and
J. H. E. T. Meuwissen. 1985. Sequential expression of antigens on sexual stages of Plasmodium falciparum accessible to transmission-blocking antibodies in the mosquito. Journal of Experimental Medicine 162:1460-1476.
Weiss, W. R., M. Sedegah, R. L. Beaudoin, L. H. Miller, and M. F. Good. 1988. CD8+ T cells (cytotoxic/suppressors) are required for protection in mice immunized with malaria sporozoites. Proceedings of the National Academy of Sciences of the United States of America 85:573-576.
Weiss, W. R., S. Mellouk, R. A. Houghten, M. Sedegah, S. Kumar, M. F. Good, J. A. Berzofsky, L. H. Miller, and S. L. Hoffman. 1990. Cytotoxic T cells recognize a peptide from the circumsporozoite protein on malaria-infected hepatocytes. Journal of Experimental Medicine 171:763-773.
Wirtz, R. A., R. Rosenberg, J. Sattabongkot, and H. K. Webster. 1990. Prevalence of antibody to heterologous circumsporozoite protein of Plasmodium vivax in Thailand. Lancet 336:593-595.
Zavala, F., A. H. Cochrane, E. H. Nardin, R. S. Nussenzweig, and V. Nussenzweig. 1983. Circumsporozoite proteins of malaria parasites contain a single immunodominant region with two or more identical epitopes. Journal of Experimental Medicine 157:1947-1957.
Zavala, F., A. Masuda, P. M. Graves, V. Nussenzweig, and R. S. Nussenzweig. 1985. Ubiquity of the repetitive epitope of the CS protein in different isolates of human malaria parasites. Journal of Immunology 135:2790-2793.